Neuropeptides are small proteins or polypeptides that serve as neurotransmitters in the nervous system generally acting via G protein–coupled receptors. Other signaling peptides such as growth factors and cytokines are considered to be distinct even though they may have some overlapping functions.
Like the monoamines and acetylcholine, neuropeptide transmitters serve primarily modulatory roles in the nervous system.
The synthesis of neuropeptides, like that of all proteins, requires the transcription of DNA and translation of the resulting messenger RNA (mRNA) into protein.
Neuropeptides are synthesized as large precursor prepropeptides that undergo extensive posttranslational processing, which includes cleavages into smaller peptides and enzymatic modification. The “pre” refers to an N-terminal signal sequence that directs newly synthesized peptides into the regulated secretory pathway.
As a result of alternative RNA splicing and differential cleavage of propeptides in different tissues, a single gene can give rise to diverse signaling peptides with distinct functions.
Unlike small-molecule neurotransmitters, which are packaged in small synaptic vesicles, neuropeptides are generally packaged in large dense core vesicles; both types of vesicles may be found in the same neuron.
Neuropeptides may diffuse for long distances within the extracellular space before binding to their specific receptors.
Neuropeptide transmitters act almost exclusively via activation of G protein–coupled receptors.
Neuropeptides and their receptors modulate many diverse functions of the central nervous system, including sleep, arousal, reward, feeding, pain, cognition, stress responses, and emotions.
Neuropeptides are short proteins or polypeptides that serve as neurotransmitters. They generally bind to G protein–linked receptors; there are rare exceptions in which peptides, such as insulin, have receptors that are enzymes (eg, protein tyrosine kinases) and act in a neurotransmitter-like fashion. Stimulation of G protein–coupled receptors produces slower responses than stimulation of ligand-gated channels (Chapter 4). Moreover, many of the actions mediated by G proteins and second messengers alter the response properties of neurons resulting in “modulation” rather than simple excitation or inhibition. More than 100 neuropeptide transmitters are known; they play diverse roles in the nervous system, including regulation of sleep and arousal, emotion, reward, feeding and energy balance, pain and analgesia, and learning and memory. However, much remains to be discovered about neuropeptide function because selective agonists and antagonists are still lacking for many neuropeptide receptors.
This chapter focuses on general aspects of neuropeptide synthesis, release, and action, and describes several neuropeptides in more detail to illustrate these concepts. Several neuropeptides are discussed at greater length in other chapters; for example, hypothalamic peptides are considered in conjunction with the regulation of neuroendocrine function, stress responses, and feeding behavior (Chapter 10), and opioid peptides are considered in connection with pain (Chapter 11). Orexin (hypocretin) peptides are described in Chapter 6 because of their widely projecting organization in the nervous system and in Chapter 13 in relation to sleep and arousal.
Peptides are small proteins or polypeptides and thus composed of amino acids covalently linked by peptide bonds 7–1. The term neuropeptide is reserved for small proteins or polypeptides that act as neurotransmitters in the nervous system. Other signaling peptides in the nervous system, such as growth factors and cytokines (Chapter 8), are generally distinguished from the neuropeptides even though they may have overlapping functions. Neuropeptides are found within the central nervous system and in the periphery, including both the sympathetic and parasympathetic nervous systems (Chapter 9). In addition to their neurotransmitter functions, some neuropeptides (eg, oxytocin and vasopressin) are released directly into the blood by neurons and act as hormones (Chapter 10); others (eg, luteinizing hormone) act as hormones secreted by endocrine glands; yet others (eg, cholecystokinin) act within peripheral organs such as the digestive system. A sampling of neuropeptides is listed in 7–1.
7–1
Peptide bond. Peptides are linear polymers composed of amino acids. The amino acids are joined by peptide bonds that result from the free carboxy group (C terminus) of one amino acid forming an amidoester bond with the free amino group (N terminus) of an adjacent amino acid. The 20 amino acids that form proteins differ from each other based on their side chains (denoted R1 and R2 in the figure). See Chapter 4 for further information.

|
|
|
|
|
|
|
|
|
|
|
|
|
|
|
|
|
|
In contrast to small-molecule neurotransmitters, the synthesis of neuropeptides, like that of all proteins, requires the transcription of DNA into messenger RNA (mRNA) and translation of mRNA into protein 7–2. A striking aspect of neuropeptide synthesis is that many steps can be regulated so that a single gene can give rise to diverse neuropeptides in a tissue-specific manner.
7–2
Synthesis of a neuropeptide. Neuropeptide synthesis is a multistep process that begins with (1) nuclear transcription of the gene that encodes one or more prepropeptides in the nucleus, splicing of the resulting primary RNA transcript to produce a messenger RNA (mRNA; described in detail in 7–5), and (2) transport of the mRNA into the cytoplasm where it is translated by ribosomes in the rough (ribosome-studded) endoplasmic reticulum (ER). (3) The N terminus of the growing prepropeptide is translated first, and contains a signal sequence that targets the growing peptide to the lumen of the ER and thence to the regulated secretory compartment. The signal sequence is cleaved by a signal peptidase even before the entire peptide is translated to yield (4) a propeptide that must undergo further enzymatic modification as required to produce active peptides.

Diversity can be generated by alternative splicing of primary RNA transcripts to produce different mRNAs (Chapter 4). Thus, for example, calcitonin and calcitonin gene–related peptide (CGRP) are generated in a tissue-specific fashion by alternative splicing of a single primary transcript to yield peptides with very different biologic actions. Calcitonin is produced in the parafollicular “C” cells of the thyroid gland and plays a central role in Ca2+ and phosphorus metabolism. CGRP is produced in neurons and, among its other actions, is a potent vasodilator, which has made its receptor a potential target for treating migraine (Chapter 20). The tachykinin family (the members of which share a C-terminal sequence) provides another example of alternative splicing of neuropeptide-encoding mRNAs. There are two genes that encode tachykinins in humans, preprotachykinin (PPT) A and B. PPT-A is alternatively spliced and, as a result, encodes three distinct prepropeptides that give rise to multiple peptides, including substance P and neurokinin A (NKA) 7–3. PPT-B gives rise to neurokinin B (NKB).
7–3
Alternative splicing of the preprotachykinin-A (PPT-A) gene. This gene, also called PPT-I gene or substance P–neurokinin A gene, contains seven exons (numbered boxes), which are alternatively spliced into three prepropeptides (α, β, and γ PPT). The number shown above each PPT splice variant represents its amino acid length after translation. After translation and proteolytic processing, all three PPT splice variants liberate substance P, which is encoded in exon 3. Neuropeptide K is encoded in exons 3 to 6 and thus is derived only from β-PPT. Neuropeptide γ is encoded in exons 3, 5, and 6, which occur together only in γ-PPT. Neurokinin A and the neurokinin A fragment (3–10) can be synthesized from either β- or γ-PPT. (Adapted with permission from Helke CJ, Krause JE, Mantyh PW, et al: Diversity in mammalian tachykinin peptidergic neurons: multiple peptides, receptors, and regulatory mechanisms. FASEB J. 1990;4(6):1606–1615.)

Following splicing, the mature mRNA is exported to the cytoplasm for translation. The initial protein product translated on the ribosome is not an active signaling molecule, but a precursor polypeptide (a prepropeptide) that undergoes processing that converts it into one or more neuropeptides. Prepropeptides contain an N-terminal signal sequence (or “pre” sequence) that directs the newly synthesized protein into the lumen of the rough endoplasmic reticulum (ER) and thus into the proper, regulated secretory pathway 7–2. The signal sequence is cleaved by a signal peptidase even before translation is completed, described as cotranslational processing. The removal of the signal sequence results in a propeptide that is released from the ribosome after translation is complete, transferred to the Golgi complex, and subsequently packaged within large dense core vesicles (LDCVs). Within the Golgi complex and within LDCVs, the propeptide undergoes additional posttranslational processing, which involves additional cleavages and covalent modifications.
Different patterns of tissue-specific cleavage are another step by which a diversity of signaling peptides can be generated. Posttranslational processing can be illustrated by the cleavage and modification of proopiomelanocortin (POMC; 7–4), the precursor of several peptides with distinct biologic actions, including adrenocorticotropic hormone (ACTH), α-melanocyte-stimulating hormone (α-MSH; also called melanocortin), and β-endorphin.
7–4
Proteolytic processing of proopiomelanocortin (POMC). After the signal peptide is removed from pre-POMC, the remaining propeptide undergoes an ordered process of endoproteolysis by prohormone convertases 1 and 2 (PC1 and PC2) at dibasic residues. PC1 is involved in the early steps of POMC processing and liberates the bioactive peptides adrenocorticotropic hormone (ACTH), β-endorphin (β-End), and γ-lipotropic hormone (γ-LPH). PC2 cleaves ACTH into corticotropin-like intermediate lobe peptide (CLIP) and α-melanocyte-stimulating hormone (α-MSH) and also releases γ-MSH from the N-terminal portion of the propeptide. The joining peptide (JP) is the region of the precursor between ACTH and γ-MSH. Some of the resulting peptides are amidated or acetylated before they become fully active (see also 7–2).

The endoproteases, prohormone convertases 1 and 2 (PC1 and PC2), which make the initial cleavages within propeptides, do so at dibasic amino acid pairs (Lys–Arg, Lys–Lys, Arg–Arg, or Arg–Lys). The resulting peptides are further modified by exopeptidases, which remove the free N- and C-terminal Lys or Arg 7–2. In addition to the action of proteases, many neuropeptides are further modified. N-terminal acetylation of α-MSH significantly enhances its biologic activity; conversely, acetylation of β-endorphin reduces its activity. Peptides with a C-terminal glycine, such as α-MSH, may also undergo α-amidation.
Enzyme | Function |
---|---|
Prohormone convertases 1 and 2 (PC1, PC2) | Endoproteolysis between dibasic amino acid residues (Lys and/or Arg) |
Carboxypeptidase E | Removes carboxyterminal basic residues exposed by cleavages |
Aminopeptidases | Removes N-terminal basic residues exposed by cleavages |
Peptidyl glycine α-amidating monooxygenase (PAM) | Amidates C-terminal glycine residues |
N-Acetyltransferases | Acetylates N-terminal amino acids of some peptides |
Signal peptidase | Cleaves N-terminal signal sequence |
There is an interesting and successful example of a peptide-processing enzyme serving as a drug target: inhibitors of angiotensin-converting enzyme (ACE), also known as peptidyl dipeptidase A, such as captopril, enalapril, and lisinopril, are widely used to treat hypertension. ACE cleaves the decapeptide angiotensin I to yield the far more active octapeptide, angiotensin II. ACE is also involved in inactivation of an additional neuropeptide, bradykinin. Because angiotensin II is a potent vasoconstrictor and bradykinin is a vasodilator, ACE inhibitors decrease blood pressure. This example may not be generalizable to enzymes such as PC1 and PC2, because they act on a very large number of neuropeptides and would thus lack specificity.
Neuropeptides are processed and stored in LDCVs, which are assembled in the Golgi apparatus and transported to the synapse. In contrast, glutamate, γ-aminobutyric acid (GABA), and other small-molecule neurotransmitters are stored in small clear synaptic vesicles (SSVs), which can be assembled in synaptic terminals. In central neurons, monoamines (eg, norepinephrine, dopamine, and serotonin) are largely stored in SSVs, although they are stored in LDCVs in adrenal chromaffin cells and various cultured cell lines. LDCVs containing peptides commonly coexist with SSVs containing small-molecule neurotransmitters. A few examples of neuropeptides and small-molecule neurotransmitters that are colocalized to the same terminals are given in 7–3. There are also examples of multiple peptides being colocalized within the same neurons. One striking example is some neurons of the supraoptic nucleus of the hypothalamus that may contain oxytocin, enkephalin, dynorphin, cholecystokinin, and cocaine- and amphetamine-regulated transcript (CART).
Neuropeptides | Small Molecule | Sites of Colocalization |
---|---|---|
Neuropeptide Y (NPY) | Norepinephrine | Locus ceruleus neurons; sympathetic preganglionic neurons |
VIP | Acetylcholine | Parasympathetic preganglionic neurons |
CGRP | Acetylcholine | Spinal motor neurons |
Neurotensin, cholecystokinin | Dopamine | Substantia nigra neurons |
TRH, substance P, enkephalin | Serotonin | Raphe nuclei neurons |
Enkephalin | GABA | Striatal neurons projecting to the globus pallidus |
Dynorphin, substance P | GABA | Striatal neurons projecting to the substantia nigra pars reticulata |
Although LDCVs and SSVs can be colocalized to the same terminals, their contents are released by different mechanisms, and indeed in response to different types of stimulation. SSVs are clustered in active zones abutting the synaptic cleft (Chapter 3). LDCVs appear to be excluded from these zones and are found at greater distances from the synapse 7–5. The exocytosis of SSVs occurs in response to large, transient increases in intracellular Ca2+, whereas the exocytosis of LDCVs requires increases in Ca2+ of lesser magnitude but longer duration, so that the Ca2+ can diffuse in adequate concentrations to reach these vesicles. Typically, a single action potential can cause SSVs to fuse with the cell membrane, but a rapid train of action potentials may be required to trigger release of neuropeptides from LDCVs. Thus, specific patterns of electrical activity in a neuron may lead to the preferential release of a neuropeptide or a small-molecule neurotransmitter, or may prompt the release of both. Because neuropeptides tend to be released under conditions of sustained activity, they may regulate strongly stimulated synapses by providing positive or negative feedback.
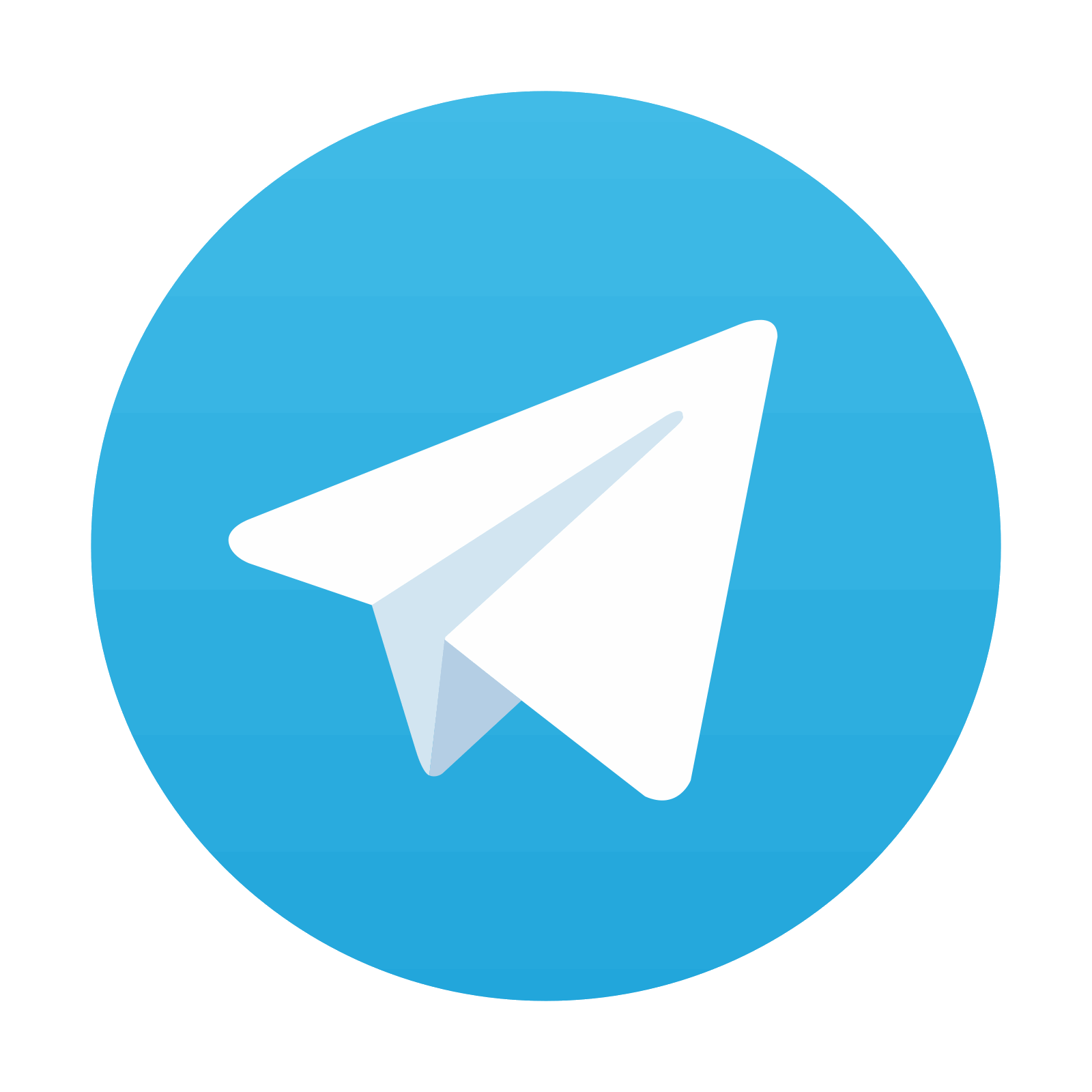
Stay updated, free articles. Join our Telegram channel
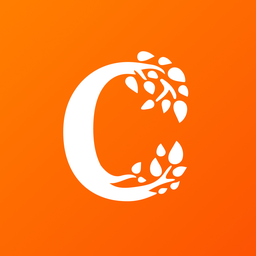
Full access? Get Clinical Tree
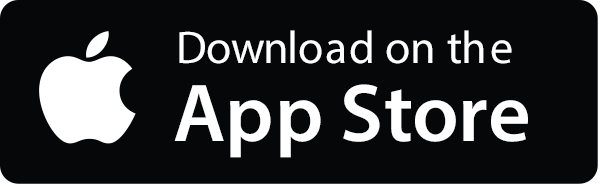
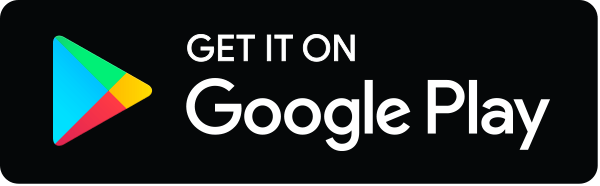