Figure 6.1. Morphology and histology of neuronal and glial elements in the neocortex. Rectangles and arrows indicate extended sections. In section 1, only a minor portion of the neurons is stained. A, axon; D, dendrite; G, glial cell; S, synapse. (Modified from Gaze RM. The Formation of Nerve Connections. New York: Academic Press; 1970; Purpura DP. Dendritic differentiation in human cerebral cortex: normal and aberrant developmental patterns. In: Kreutzberg GW, ed. Advances in Neurology. Vol. 12. New York: Raven Press; 1975:91–116; Valverde F. The organization of area 18 in the monkey: a golgi study. Anat Embryol. 1978;154:305–334; Westrum LE, Blackstad TW. An electron microscopic study of the stratum radiatum of the rat hippocampus (regio superior, CA1) with particular emphasis on synaptology. J Comp Neurol. 1962;113:281–293, with permission.)
Neurons
A typical neuron consists of a soma (body, perikaryon) and fibers (dendrites and axons). In functional terms, with respect to information input, the relatively short and highly arborized dendrites can be considered extensions of the soma, as reflected in their being covered by thousands of synaptic endings. Axons are relatively long and, especially in their terminal regions, branch into collaterals. These neuronal output structures carry information into the terminal regions. Information is transferred to other neurons by way of synaptic endings (1–9).
Neuronal function is closely correlated with bioelectrical activity, which can be studied with intracellular microelectrode recordings. When a neuron is impaled by a microelectrode, a membrane potential of approximately 70 mV with negative polarity in the intracellular space becomes apparent. This resting membrane potential, existing in the soma and all its fibers, is based mainly on a potassium outward current through leakage channels. If the resting membrane potential is critically diminished, that is, if a threshold is surpassed, an action potential (AP) is triggered, which is based on sodium inward and potassium outward currents through voltage-dependent membrane channels. APs are conducted along the axons to the terminations, where they lead to a release of transmitter substances at the chemical synapse. These transmitters open another class of membrane channels in the postsynaptic neuron. Dependent on the ionic composition of the currents flowing through the transmitter (ligand)-operated channels, two types of membrane potential changes, commonly called postsynaptic potentials (PSPs), are induced in the postsynaptic neuron. When a sodium inward current prevails, depolarization of the postsynaptic neuron occurs. This synaptic depolarization is called an excitatory postsynaptic potential (EPSP) because it increases the probability that an AP will be triggered. When a potassium outward current or a chloride inward current prevails, hyperpolarization of the postsynaptic neuron occurs. Because hyperpolarization increases the distance between membrane potential and threshold, the synaptic hyperpolarization is called an inhibitory postsynaptic potential (IPSP) (10–12).
The EPSPs and IPSPs can interact with each other (Fig. 6.2). Electrical stimulation of an axon (ST1 in Fig. 6.2A) forming an excitatory synapse on a postsynaptic neuron can induce an AP at the site of stimulation. Conducted along the axon, the AP finally induces an EPSP in the postsynaptic neuron (ST1 in Fig. 6.2B). When only one synapse separates the site of stimulation from the site of EPSP generation, a monosynaptic EPSP appears. One way in which a summation of EPSP takes place is when the stimulation is repeated with an interstimulus interval shorter than the duration of the EPSP. With this temporal summation, the second EPSP can surpass the threshold and induce an AP (ST1 in Fig. 6.2B). A summation of EPSPs also can occur when monosynaptic EPSPs are evoked simultaneously at several locations on the postsynaptic neuron (spatial summation). Temporal and spatial summations are often combined with each other and are essential for information processing in the central nervous system, as when the AP reaches the target neuron by different ways. With stimulation at ST2 in Figure 6.2A, the triggered APs pass through varying numbers of relays before reaching their target. As APs are delayed with each synaptic transmission, they appear with temporal dispersion at the postsynaptic neuron and induce a long- lasting depolarization (ST2 in Fig. 6.2B). Because many synapses are involved, such a depolarization is called a polysynaptic EPSP. When a polysynaptic network is activated repeatedly, EPSPs of considerable amplitude and duration can appear, as demonstrated by the original recording in Figure 6.2C. As with EPSPs, IPSPs can be induced both monosynaptically and polysynaptically and also are subject to temporal and spatial summation (ST3 and ST4 in Fig. 6.2A and B) (5,11,12).
Figure 6.2. Bioelectrical activity of neuronal elements: membrane potential (MP), action potential (AP), excitatory postsynaptic potential (EPSP), and inhibitory postsynaptic potential (IPSP). A:Indicated are stimulation sites and the pyramidal neuron from which the recording was made. Open symbols represent excitatory synapses and filled symbols inhibitory synapses. Up to four interneurons are schematically drawn between stimulation sites (ST1 to ST4) and the neuron. B:Intracellular recording from the pyramidal neuron in (A) is shown. Single electrical stimuli applied at ST1 and ST3 evoked monosynaptic EPSP and IPSP, respectively. Paired stimulation at ST1 and ST3 led to a summation of the corresponding monosynaptic responses. After stimulation at ST2 and ST4, polysynaptic EPSP and IPSP, respectively, were elicited. C:Original tracing of synaptically mediated neuronal depolarizations in a spinal motoneuron of the cat is shown. Stimulation (ST) of pathways oligosynaptically and polysynaptically linked to the neuron led to early (oligosynaptic) and late (polysynaptic) potentials. (A and B adapted from Speckmann E-J. Experimentelle Epilepsieforschung. Darmstadt, Germany: Wissenschaftliche Buchgesellschaft; 1986:13, with permission.)
In complex neuronal systems, EPSPs and IPSPs are often superimposed and induce long-lasting sequences of fluctuations of the membrane potential. These kinds of postsynaptic responses play a prominent role in the generation of extracellular potential fields, such as the EEG.
Glial Cells
Consisting of a soma and fibers, glial cells intermingle with the neuronal structures. Glial cell fibers are electrically coupled, building up an extended functional network (3,8,13).
Glial cells also show a membrane potential (Fig. 6.3A). Unlike neurons, glial cells do not generate APs and PSPs. Because their resting membrane potential is based exclusively on potassium outward current through leakage channels, its value is close to the potassium equilibrium potential. With an increase and a subsequent decrease in extracellular potassium concentration, glial cells depolarize and repolarize, respectively (Fig. 6.3A). Changes in the extracellular concentration of other cations have only small effects on the membrane potential of glial cells (14,15).
Figure 6.3. Changes in membrane potential (MP) of a glial cell induced by an increase in extracellular potassium concentration (A) and functional linkage between neuronal and glial activity (B) and (C). A: The increased extracellular concentration of K+ led to a sustained depolarization of the glial cell. B: During a neuronal action potential, an efflux of K+ occurred. C: The K+ concentration in the extracellular space close to the glial cell was raised during the repetitive firing of a neuron. This led to sustained depolarization of the neighboring glial cell. (A and C adapted from Zenker W. Feinstruktur des Nervengewebes. In: Zenker W, ed. Makroskopische und Mikroskopische Anatomie des Menschen. Vol. 3. Munich, Germany: Urban & Schwarzenberg; 1985:3–55, and B and C adapted from Valverde F. The organization of area 18 in the monkey: a golgi study. Anat Embryol. 1978;154:305–334.)
Glial cells and neurons are functionally linked by way of the extracellular potassium concentration (Fig. 6.3B and C). As mentioned, neuronal APs are associated with an outflow of potassium ions (see Fig. 6.3B). Thus, with an increase in the repetition rate of neuronal APs, the extracellular potassium concentration increases, resulting in depolarization of glial cells adjacent to the active neurons (see Fig. 6.3C) (11,14–16).
PRINCIPLES OF FIELD POTENTIAL GENERATION
Changes in membrane potential of neurons and glial cells are the basis of changes in extracellular field potential. The mechanisms involved can be described as follows: (i) primary transmembranous ion fluxes at a restricted membrane area of cells and consequent localized membrane potential changes; (ii) development of potential gradients between sites of primary events and the remaining areas of the membrane; and (iii) secondary ion currents because of the potential gradient along the cell membrane in the intracellular and extracellular spaces. The secondary current flowing through the extracellular space is directly responsible for the generation of field potentials (9,17). Because EPSPs and IPSPs are important in the generation of the EEG findings, the processes are explained in greater detail using the examples of an excitatory synaptic input (2,12,18,19).
A vertically oriented neuronal element, shown schematically in Figure 6.4, is impinged on by a single excitatory synapse whose afferent fiber can be stimulated. The resulting net influx of cations leads to depolarization of the membrane, that is, to an EPSP. Consequently, a potential gradient exists along the neuronal membrane and evokes an intracellular and extracellular current flow. As a result of the intracellular current, the EPSP spreads electrotonically; the extracellular current induces field potentials. The polarity depends on the site of recording. The electrode near the synapse “sees” the inflow of cations (a negativity), whereas the electrode distant from the synapse “sees” the outflow of cations (a positivity). Between the two electrodes is the reversal point of the field potentials (12,20).
Figure 6.4. Principles of field potential generation in the neocortex. A perpendicular pyramidal neuron with an extended intracellular space (hatched area) is shown. An afferent fiber (left) formed an excitatory synaptic contact at the superficial aspect of the apical dendrite. Changes in membrane potential and in corresponding field potential are given in the intracellular and extracellular spaces, respectively. After stimulation of the afferent fiber (ST), an excitatory postsynaptic potential developed in the upper part of the dendrite and spread electrotonically to the lower parts. The local excitation (+ and −) led to tangential current flows (broken lines) and to the field potential changes in the extracellular space.
Corresponding effects occur with the generation of IPSPs. Activation of an inhibitory synapse induces an outflow of cations or an inflow of anions at the synaptic site. In this way, the membrane potential is increased at the synaptic site, and a potential gradient develops along the cell membrane, similar to that described for EPSPs. The potential gradient evokes a current flow from the synaptic site to the surrounding regions of the membrane. Compared with EPSPs, the extracellular current flow is inverted, as is the polarity of field potentials. Thus, the electrode near the synapse “sees” a positivity and the electrode distant from the synapse a negativity.
Field potentials are generated by extracellular currents, and their polarity depends on the direction of the current as well as on the positions of the extracellular electrodes. Figure 6.5 illustrates the generation and polarity of field potentials, as elicited by excitatory and inhibitory inputs to superficial and deep regions of vertical neuronal elements. Negative field potentials at the cortical surface may be based on superficial EPSPs as well as on deep IPSPs, and positive field potentials at the surface may be based on superficial IPSPs as well as on deep EPSPs (Fig. 6.6) (12,19,20).
Figure 6.5. Generation of field potential in the neocortex by excitatory and inhibitory synaptic inputs reaching the superficial and deep parts of perpendicular pyramidal neurons. The intracellular space is extended (hatched areas). Changes in membrane potential and in the corresponding field potential are given in the intracellular and extracellular spaces, respectively. Locations of active inputs are indicated (heavy arrows). EPSP and IPSP, excitatory and inhibitory postsynaptic potentials, respectively. Excitatory inputs: With superficial excitation, an inward current generated an EPSP in upper and lower regions. Because of the direction of the extracellular current flow (light arrows), the field potential had negative polarity at the surface and positive polarity in the deep recording (cf. Fig. 6.4). With deep excitation, the current flow—and the field potentials—had inverse direction to that elicited by superficial excitation. Inhibitory inputs: With deep inhibition, an outward current generated an IPSP in lower and upper regions. Because of the direction of the extracellular current flow (arrows), the field potential had positive polarity in the deep recording and negative polarity at the surface. With superficial inhibition, the direction of current flow was inverse to that seen with deep inhibition; the field potentials were inverted as well. Differences in the shape of the various potentials were caused by the electrical properties of the tissue.
Figure 6.6. Synopsis of the synaptic processes underlying the generation of superficial field potentials in the cerebral cortex. Different mechanisms may lead to uniform superficial field potentials.
POTENTIAL FIELDS IN NEURONAL NETWORKS
Many neuronal elements contribute to the extracellular currents that generate field potentials recorded at the surface of central nervous system structures. The spatial arrangement of the neuronal elements and the positions of the recording electrodes play an essential role in establishing and detecting extracellular potential fields (2,12,21).
Two principal types of neuronal arrangements can be identified (Fig. 6.7). In the parallel type, the somata are in one layer and the dendrites are in opposite layers (see Fig. 6.7A). In the other type, the somata are in the center of a pool and the dendrites extend to its periphery (see Fig. 6.7B). The first arrangement is realized in the cortex and the second in brainstem nuclei.
Figure 6.7. Neurons arranged to give open (A) and closed (B) fields. Field potentials are present (A) or missing (B) during excitatory inputs by way of afferent fibers. E1 and E2 indicate different and reference electrodes.
The two neuronal arrangements build up the so-called open and closed fields. In open fields, one electrode (E2 in Fig. 6.7A) largely integrates the potentials of the population (i.e., it is near the zero potential line), and the other electrode (E1 in Fig. 6.7A) sees only the positive or negative field, permitting the recording of a field potential. In closed fields, external electrodes do not see significant potential differences because the current flows within the pool compensate for each other (see Fig. 6.7B) (2,21).
TYPES OF FIELD POTENTIAL CHANGES
With respect to the time course, two types of field potentials can be differentiated, depending on the time constant of the amplifying recording device. The conventional EEG is recorded with a time constant of 1 second or less. Amplification with an infinite time constant, that is, by a DC amplifier, permits additional recording of baseline shifts and wavelike potentials (EEG/DC) (22–24).
Wave Generation (Conventional Electroencephalogram)
The generation of wavelike potentials is described in Figure 6.8, a representation of a column of neocortex. In its upper dendritic region, the neuron is activated by an afferent fiber by way of an excitatory synapse. The superficial EEG and the membrane potentials of the dendrite and afferent fiber are recorded. The afferent fiber shows grouped, followed by regular, discharges. With grouped discharges prominent, summated EPSPs occur in the dendrite; with sustained regular activity, a depolarizing shift of the membrane potential appears. The changes in membrane potential in the upper dendrite lead to field potentials. When amplifiers with a finite time constant are used, only fluctuations in field potential are recorded, corresponding to findings on conventional EEG. The shift of the membrane potential is not reflected (21,25).
Figure 6.8. Wave generation in the electroencephalogram (EEG) at the surface of the cerebral cortex. A perpendicular pyramidal neuron is shown. An afferent fiber formed an excitatory synaptic contact at the superficial part of the apical dendrite. Simultaneous recordings of the membrane potentials (MPs) of the afferent fiber and the dendritic element, as well as of the EEG, are displayed. Groups of APs in the afferent fiber generate wavelike excitatory postsynaptic potentials (EPSPs) in the dendritic region and corresponding waves in the EEG recording. Tonic activity in the afferent fiber results in long-lasting EPSP with only small fluctuations. The long-lasting depolarization is not reflected on the conventional EEG recording.
Baseline Shifts (Electroencephalogram/Direct Current)
The generation of baseline shifts is described in Figures 6.9 and 6.10. In a column of the neocortex (see Fig. 6.9), a neuron is activated in its upper dendritic region by an afferent fiber by way of an excitatory synapse. In this case, the afferent fiber displays three levels of sustained activity. Medium regular activity is interrupted by periods of high repetition and silence. Consequently, owing to facilitation, the upper dendrite is depolarized during the high discharge in the afferent fiber and is hyperpolarized in the silent period because of disfacilitation. This results in corresponding field potential shifts. When amplifiers with an infinite time constant are used, these baseline shifts, which reflect sustained values of the membrane potential of neuronal elements, are recorded. With a sufficiently high upper frequency limit, the DC recording comprises conventional EEG waves as well as slow potential deviations (26–32).
Figure 6.9. Sustained shifts in the electroencephalogram (EEG) at the surface of the cerebral cortex resulting from sustained neuronal activities. If recordings are performed with a direct current (DC) amplifier (EEG/DC), sustained potentials can also be recorded at the surface. In the perpendicular pyramidal neuron depicted, an afferent fiber formed an excitatory synaptic contact at the superficial part of the apical dendrite. The membrane potentials (MPs) of the afferent fiber and the dendritic element were recorded simultaneously, as was the EEG/DC. Increased and decreased sustained activity in the afferent fiber generated sustained depolarizations and hyperpolarizations of the dendritic region and corresponding negative and positive shifts of the EEG/DC recording.
Figure 6.10. Sustained shifts in the electroencephalogram (EEG) performed with a direct current (DC) amplifier (EEG/DC) at the surface of the cerebral cortex generated by neuronal activity and mediated by a glial network. If recordings are performed with a DC amplifier, sustained potentials can also be recorded. A deep neuron functionally coupled to a perpendicularly oriented glial network is shown. The membrane potentials (MPs) of the deep neuron and of a glial cell as well as the EEG/DC were recorded simultaneously. Sustained increased activity of the deep neuron induced an increase in extracellular K+ concentration and a corresponding depolarization of the glial cells. Because of the electrotonically coupled network of glial cells, a sustained positive potential was induced in the surface EEG/DC recording.
Glial cells also are involved in the generation of baseline shifts (Figs. 6.10 and 6.11). As noted, a functional coupling between neurons and glial cells exists (see Fig. 6.3). Figure 6.10 shows a neuron in deep cortical layers and a network of electrically coupled glial cells extending to the surface. The superficial EEG/DC and the membrane potentials of a glial cell and the neuron are recorded. With increased discharge frequency of the neuron, extracellular potassium concentration rises, evoking a depolarization of the adjacent glial cell. The potassium- induced depolarization is conducted electrotonically within the glial network. A functional situation is present similar to that in a perpendicular neuron with a deep excitatory synaptic input (see Figs. 6.5 and 6.6). The superficial EEG/DC electrode sees a long-lasting positivity because of an outflow of cations from the glial cells in the upper layers. In other respects, this corresponds to the well-known spatial buffering of potassium. In principle, the aforementioned mechanism can make visible the activity of closed fields (see Fig. 6.7B) in baseline shifts of field potentials (31,33,34).
Figure 6.11. Simultaneous recordings of the electroencephalogram (EEG) potential performed with a direct current (DC) amplifier (EEG/DC) at the surface of the cerebral cortex and of the membrane potential (MP) of glial cells in an anesthetized and artificially ventilated rat. A:High-frequency electrical stimulation of the cortical surface (horizontal bar) is indicated. B:Focal epileptic activity induced by penicillin is indicated. Repetitive cortical stimulation (horizontal bar) increased the frequency of epileptic discharges (interruption, approximately 5 seconds). C:Increase of the local partial pressure of carbon dioxide (PCO2) during apnea (horizontal bar) is shown. ST1 and ST2, low- and high-frequency electrical stimulation of the cerebral cortex, respectively. Depolarization of glial cells can be associated with both a positive (C) and a negative (A and B) shift in the EEG/DC. (A adapted from Caspers H, Speckmann E-J, Lehmenkühler A. DC potentials of the cerebral cortex. Seizure activity and changes in gas pressures. Rev Physiol Biochem Pharmacol. 1987;106:127–178; B adapted from Speckmann E-J. Experimentelle Epilepsieforschung. Darmstadt, Germany: Wissenschaftliche Buchgesellschaft; 1986; and C adapted from Caspers H, Speckmann E-J, Lehmenkühler A. Electrogenesis of slow potentials of the brain. In: Elbert T, Rockstroh B, Lutzenberger W, et al., eds. Self-regulation of the Brain and Behavior. New York: Springer; 1984:26–41, with permission.)
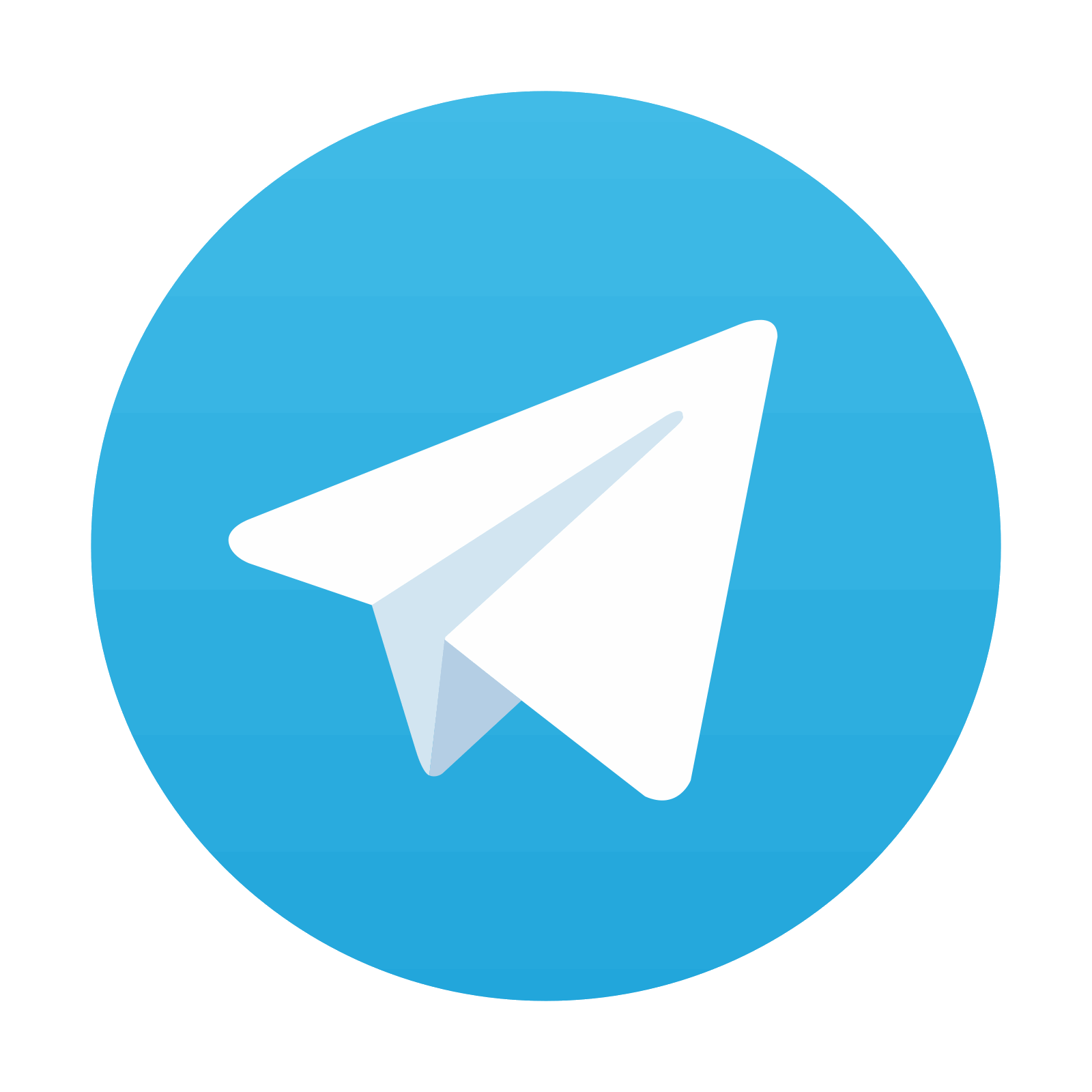
Stay updated, free articles. Join our Telegram channel
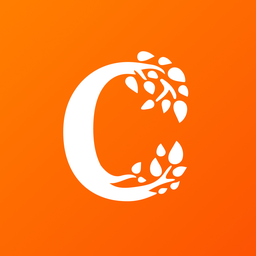
Full access? Get Clinical Tree
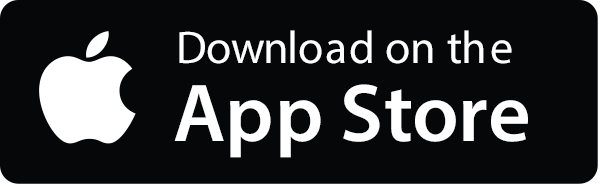
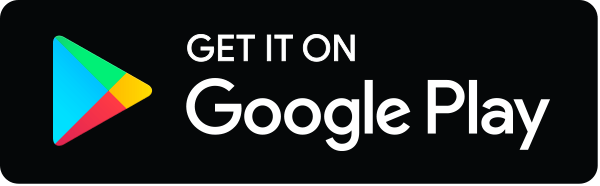