Chapter 23 Normal Physiology of the Upper and Lower Airways
Abstract
Chapters 21 and 22 described the basis of the physiology of respiratory function during wake and sleep. In this chapter we focus on the physiologic determinants of respiration used to estimate the breathing function in normal persons. The ultimate goal is to allow readers who are not familiar with the field of respiratory medicine to benefit from the more subtle concepts that will help them to better understand sleep-disordered breathing.
The aim of breathing is to provide oxygen to the different body parts and to eliminate carbon dioxide resulting from cell metabolism. This is achieved through continuous gas exchange between inspired and exhaled air and the blood in the pulmonary circulation. Once blood coming from the right heart has been loaded with oxygen, it reaches the left heart, which sends it to every part of the body through the arterial system. The different organs then take up oxygen from the arterial blood and remove carbon dioxide. Blood loaded with carbon dioxide travels through the venous system to reach the pulmonary circulation, where carbon dioxide passively diffuses through the alveolocapillary membrane into the airway, where it is exhaled. Survival depends upon the integrity of this physiologic process, and death can occur if this system stops for more than a few minutes. Therefore, maintenance of normal arterial blood gases involves several physiologic systems (control of breathing, thoracopulmonary mechanics, circulatory characteristics, blood-transport characteristics) that are intimately linked to one another. This chapter focus only on the mechanical properties of the chest and upper and lower airway, which are the main determinants of ventilation during sleep (Box 23-1).
Anatomy and Physiology
Breathing is possible through either the nose or the mouth, but nasal breathing is the physiologic breathing route. The lower airway includes the trachea and the lungs (bronchi and alveoli). Thin blood vessels, the capillaries lining the alveoli, allow gas exchange between inspired air and blood. The rib cage provides protection for the lungs and also allows them to change volume from a minimum of about 1.5 L to a maximum of 6 to 8 L, depending on the height and sex of the person.1
Respiratory Muscles
The diaphragm is the main muscle of respiration. It is a dome-shaped muscle that separates the thoracic and abdominal cavities. The diaphragm is innervated by the phrenic nerves. During inspiration, the neural outflow coming from the central respiratory centers leads to diaphragm contraction; the shortening of the diaphragm muscles fibers flattens the diaphragm, making it lose its dome shape and thus increasing intrathoracic volume. Intercostal muscles can also increase the intrathoracic volume by elevating ribs and increasing the anteroposterior diameter of the thorax (Fig. 23-1). Accessory breathing muscles such as the scalene or sternocleidomastoid are not active during normal breathing, but they can be recruited during an effort or in the presence of thoracopulmonary disorders.
Elastic Forces and Lung Volumes
where V is volume and P is pressure.
The lung volume, in the natural resting end-expiratory position, is its functional residual capacity (FRC). Total lung capacity (TLC) is reached when the thoracic cage and lungs are fully expanded (maximal inspiratory effort). Residual volume (RV) represents the volume remaining in the lungs at the end of a forced expiration. Vital capacity (VC) is the maximum amount of air that can be expelled after the lungs have been fully inflated. Tidal volume (VT) is the volume of air inspired or expired during each quiet breathing cycle (Fig. 23-2). The typical VT value is 500 mL, but it can dramatically increase during exercise. Only about two thirds of inspired air participates in oxygen and carbon dioxide exchange because the volume corresponding to upper airway, trachea, and bronchi do not contribute to gas exchanges; this area is the dead space (VDS) of the respiratory tract.1
Breathing Cycle and Minute Ventilation
where RR is the respiratory rate. During quiet breathing, a typical value is 6 L/min but the volume can rise to 180 L/min during exercise.
Resistance
where ΔP is the pressure difference and R is the resistance. Airflow is described as turbulent when the pressure drop along the airway is proportional to flow and its square values:
where ΔP is the pressure difference and V is the air flow. Airflow along airways is complex and usually consists of a mixture of laminar and turbulent flow. In normal lungs, respiratory resistance depends mainly on airways diameter. The velocity of airflow and airways diameter decreases in successive airways generation from a maximum in the trachea to almost zero in the smallest bronchioles.
Effects of Obesity and Body Posture on Lung Volumes
In an awake normal and healthy subject, there is a reduction in FRC and TLC in the supine position in comparison to upright body position.2 This reduction is thought to be due to an increase in intrathoracic blood volume or to the gravitational effect of abdominal contents pushing the relaxed diaphragm into a more rostral position.3 This change in diaphragm position reduces the diaphragm’s ability to contract, as suggested by a decreased maximal inspiratory pressure in the supine posture with respect to the upright and sitting positions.4 Moreover, this restrictive defect in lung volume increases the work of breathing and deteriorates gas exchange by decreasing the ventilation-perfusion ratio in the dependent parts of the lungs. Decreased lung volume can also increase upper airway resistance by reducing the caudal traction of the mediastinum and trachea on the pharyngeal walls, making them more collapsible during inspiration (discussed further later).5–8
In obese subjects, a restrictive defect in lung volume is also observed when they are sitting. There is a further small decrease of about 70 to 80 mL from about 2.4 L (for an average-sized man) in FRC and TLC when obese subjects lay supine.2 Considering the effects of abdominal volume on lung function in sitting obese subjects, one would expect a greater reduction in lung volume when they adopt the supine position compared to lean persons. However, a lesser decline in FRC and TLC in obese subjects in the supine position is reported.2,3,9 One possible explanation is that in sitting obese subjects, the diaphragm is already shifted in a more rostral position and it cannot move much farther in the supine position. Two experimental studies also suggest that there may be a protective or adaptive mechanism against large changes in end-expiratory lung volume during wakefulness10 and sleep.11
Maximal minute ventilation, expiratory reserve volume, forced vital capacity (FVC), and, to a lesser extent, forced expiratory volume in 1 second (FEV1) are also affected by obesity.12 The estimated reduction of FVC is 17.4 mL/kg weight gain for men and 10.6 mL/kg weight gain for women.13 Men show more impairment of FVC with weight gain than women, possibly because of differential patterns of fat deposition: waist circumference is negatively associated with FVC and FEV1. On average, a 1-cm increase in waist circumference was associated with a 13-mL reduction in FVC.14 All these changes observed from upright to supine positions and in obese persons may contribute to the exacerbation of respiratory disturbances in the presence of sleep-disordered breathing as described in Section 13 of this volume.
Effects of Sleep on Lung Volume
There is a modest but significant decrease in FRC occurring during sleep in most healthy subjects. FRC decreases by about 200 mL in stage 2 non–rapid eye movement (NREM) sleep and by 300 mL during slow-wave sleep and REM sleep when measured with a helium dilution technique in comparison to normal value awake (~2.4 L for an average-sized man).15 When plethysmongraphy is used to measure differences in lung volume, a 440 to 500 mL decrease in lung volume was reported in NREM sleep (stages 2 to 3 and 4), with a similar decrease in REM sleep.16 Possible mechanisms of the decrease in FRC during sleep are diaphragm hypotonia inducing rostral displacement of the diaphragm, alteration of the respiratory timing from central generator of breathing, decrease in lung compliance, decrease in thoracic compliance, and central pooling of blood (see Chapters 21 and 22).
A reduction in tidal volume by about 6% to 15% has been reported during NREM sleep (stages 2 to 4), with a further decrease during REM sleep (about 25% lower than during wake).17,18 Minute ventilation is significantly lower during all NREM sleep stages compared to wake and further decreases during REM sleep, especially during phasic REM (about 84% of the level during wake) (Fig. 23-3).17–20 This is due to a faster and shallower breathing pattern in all sleep stages with a lower tidal volume, especially during REM sleep. This is, however, controversial because another study showed no significant change in VT between wakefulness and any sleep stage and suggested that the decrease in minute ventilation (8% in NREM and 4% in REM sleep) is due to a decrease in respiratory rate.21 Most studies agree, however, that during NREM sleep there is an increase in the rib cage’s contribution to VT associated with an approximately 34% increase in the activity of intercostal muscles.20–22 There is thus an apparent contradiction between the increase in electromyogram (EMG) activity of thoracic muscles and a decrease in minute ventilation. A possible explanation is that even though muscle activity increases, the actual negative thoracic pressure decreases because of a decrease in the efficiency of muscle contraction during NREM sleep.23 During REM sleep the relative contribution of the rib cage and abdomen is not significantly different compared to wakefulness.20 Age and sex do not seem to significantly alter sleep-related changes in lung volume.
Effects of Sleep on Breathing Pattern and Blood Gases
During NREM sleep, the decrease in minute ventilation induces a drop in PaO2 of 3 to 9 mm Hg and an increase in PaCO2 and Paco2 levels ranging from 2 to 4 mm Hg.24,25 During stable NREM sleep, the breathing pattern is usually regular. However, periodic breathing with waxing and waning ventilation is commonly observed at sleep onset (unstable NREM sleep).26,27 Complete cessation of breathing for more than 10 seconds with respiratory effort (obstructive sleep apnea) or without respiratory effort (central sleep apnea) can even occur at this time in healthy persons. In these circumstances, this transient periodic breathing seems to be due to an unstable ventilatory feedback loop (loop gain). A low arousal threshold during this stage can also induce instability in the sleep–wake cycle and contribute to unstable breathing. Because of the higher CO2 set point during sleep, arousals are associated with a sudden increase in ventilation, which will then decrease CO2 level. If the CO2 level is below the apnea threshold (CO2 level below which the central respiratory drive is abolished) when sleep resumes, an apnea can occur and breathing will resume only when the CO2 level again reaches the sleep set point. The magnitude and the breathing fluctuation depend on several factors such as chemoreceptor sensitivity (controller gain), lung-to-chemoreceptor circulation delay, and the efficiency of the respiratory system in inducing changes in CO2 level (plant gain) (see also Chapter 22).28
During REM sleep, ventilation is notably variable both in amplitude and frequency. This heterogeneity seems to be directly related to the intensity of phasic activity as indicated by bursts of eye movements. Specifically, phasic REM activity, characterized by a high density of rapid eye movements and muscle twitches, seems to have an inhibitory influence on ventilation.18 Overall alveolar ventilation tends to fall by about 20% compared to wakefulness, mainly because of a fall in tidal volume.20
Upper Airway
From a mechanical point of view, the upper airway behaves as a Starling resistor, where the pharyngeal airway represents the collapsible segment and is situated between two noncollapsible structures (larynx and nasopharynx). The flow pattern depends on the forces applied inside and outside the collapsible segment. The transmural pressure gradient is the net pressure difference between all these opposite forces. The collapsing forces are represented by the negative inspiratory transmural pressure gradient and the pressure applied by upper airway tissue (Fig. 23-4). The contraction of upper airway stabilizing muscles (upper airway dilators) is the main dilating force, the other being represented by tracheal traction (see Fig. 23-4). Therefore, the amount and timing of the neuromuscular activation process of upper airway stabilizing muscles and the mechanical properties of upper airway tissues play a pivotal role in determining upper airway stability.
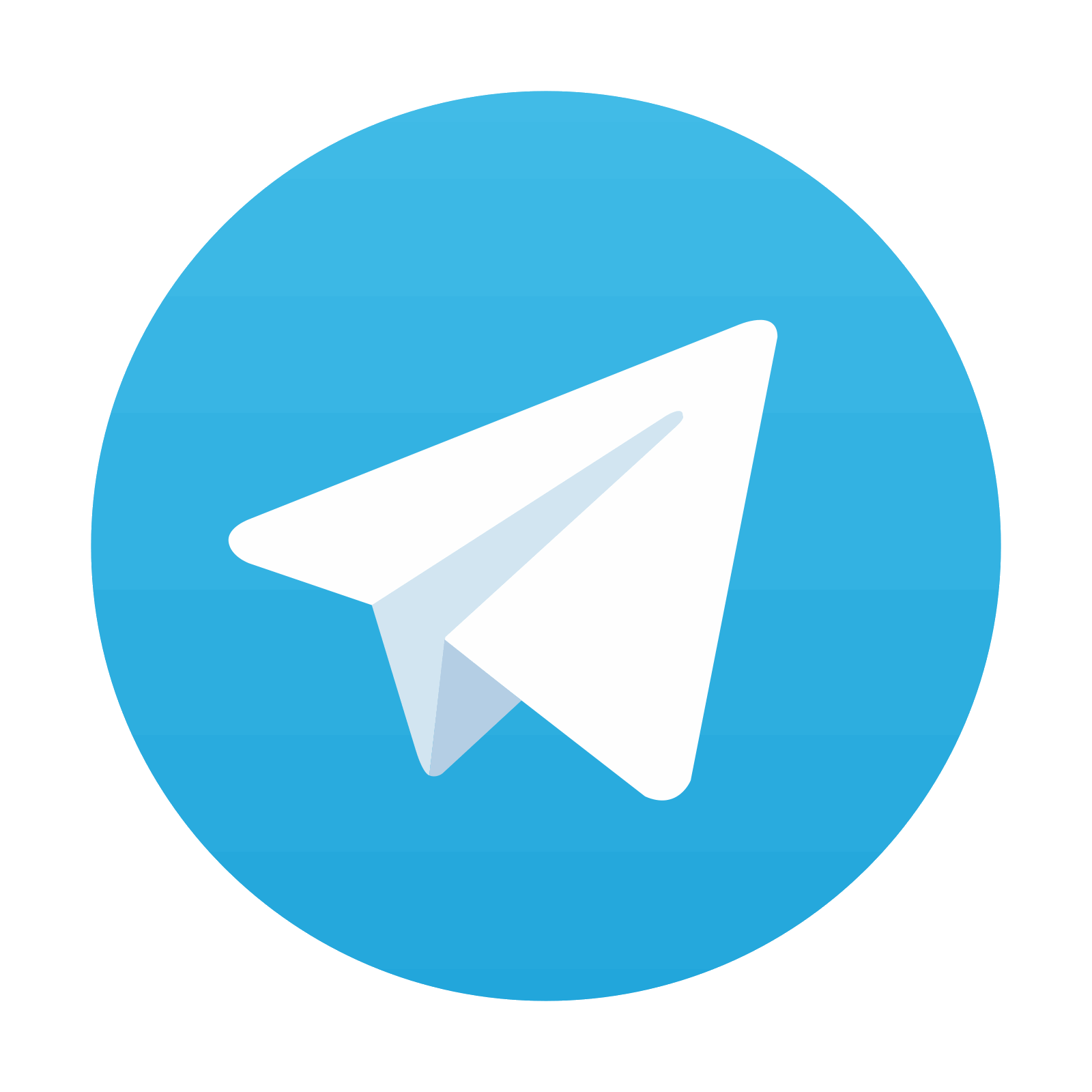
Stay updated, free articles. Join our Telegram channel
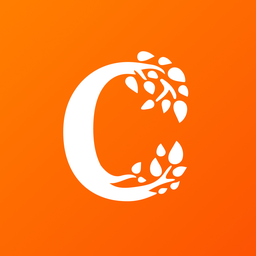
Full access? Get Clinical Tree
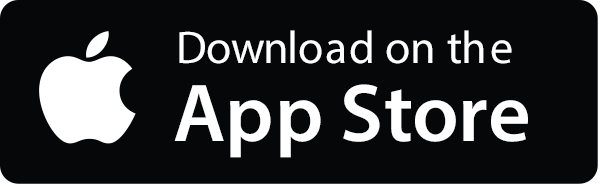
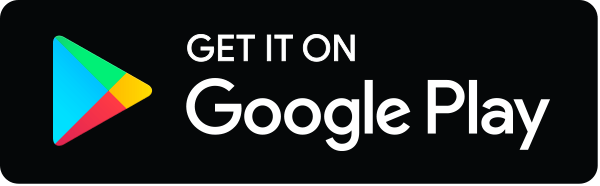