7 Perceptuo-motor control
At the end of this chapter, you should be able to:
• describe the concept of ‘coordination’ and explain the ‘degrees of freedom’ problem
• define motor control and explain motor control from an information-processing approach, critically evaluating the strengths and weaknesses of this approach
• compare and contrast the information-processing approach to motor control with a direct perception and dynamical systems approach
• explain the interaction between perception and action and explain the relevance of this interaction for rehabilitation
• explain how motor control is influenced by factors related to the individual, the task and the environment
• discuss the implications of the information presented in this chapter for health-care professionals working in rehabilitation in general, and neurological rehabilitation in particular.
Introduction
Having introduced the basic building blocks of the nervous system in Chapter 2 and explained the role of different neural circuits involved in movement in Chapter 6, the next two chapters focus on how we control and learn more complex, voluntary movements and activities.
Motor control involves the planning, initiation, execution, monitoring and adaptation of movement. Whether you are a physiotherapist, occupational therapist, speech and language therapist or orthotist, you will often play a key role in designing interventions to improve motor control, e.g. a patient’s ability to move around, engage in sporting activities, express themselves through speech or music, and participate in occupation and leisure activities. An important aim of rehabilitation is to facilitate long-term improvement in an individual’s functional activity within their own environment. The question is: how can we help patients to improve their motor control – not just within therapy sessions in the clinic – but out there, in the ‘real world’, and long after rehabilitation has ceased? A sound working understanding of motor control and learning is, therefore, essential for health-care professionals. Let us begin by considering a case study (7.1) about a person with Parkinson’s disease that illustrates a particular motor control problem and the impact this may have.
Mrs B., a 67-year-old retired music teacher, was diagnosed with Parkinson’s disease (PD) approximately 8 years ago. PD is a degenerative condition of the substantia nigra, which are part of the basal ganglia. PD is associated with a range of different signs and symptoms (see Chapter 6). This case study concentrates on Mrs B.’s particular problems with balance and gait Mrs B. has recently referred herself for domiciliary physiotherapy as she finds it increasingly difficult to move around the house, while she has also experienced an increasing number of falls. She is beginning to lose her confidence and is becoming more fearful of falling. During your history taking, Mrs B. tells you that she has particular difficulty walking across her sitting room. In contrast, moving across her kitchen seems to be much easier. You are curious to find out why Mrs B. has such difficulty in one situation, but not in the other.
Well, Purdon Martin (1967) in his remarkable book was the first to demonstrate the effect of horizontal floor markers on stride length in people with PD. Since then, many studies have demonstrated that visual cues can improve speed of walking, stride length and reduce ‘freezing’ in people with PD (Lim et al., 2005, Rochester et al. 2010). Cueing, which is defined as using ‘external temporal or spatial stimuli to facilitate movement (gait) initiation and continuation’ (Nieuwboer et al. 2007 p. 134), may involve visual (e.g. stripes, upturned walking sticks), acoustic (e.g. rhythm) or proprioceptive stimuli (e.g. vibration). Exactly how these cues affect walking is not entirely clear. Azulay et al. (1999) compared gait parameters in people with PD and normal controls under different conditions, including no stripes and transverse stripes on the floor, both under normal and stroboscopic illumination. They found that people with PD relied more than normal controls on visual information during walking, and specifically appeared to use visual information about the motion of the stripes. The authors postulated that by relying more on dedicated visuo-motor pathways people with PD were able to involve the cerebellum and bypass the affected basal ganglia circuitry during gait.
How may this research inform your practice? The implications for therapy are that the therapist can use ‘cueing’ as a therapeutic strategy to try and improve the person’s gait. Chapter 8 will present further detail from a study investigating the effects of cueing in motor learning in PD.
Coordination
Defining ‘coordination’
‘Coordination’ is a complex concept, which has fascinated scientists and clinicians for centuries (Latash, 2008, Latash and Zatsiorsky 2001). Hence, unsurprisingly, the term ‘coordination’ has been defined in numerous different ways. A ground-breaking definition was articulated by Nikolai Aleksandrovich Bernstein (1896–1966), the famous Russian physiologist and founder of motor control science, whose legacy you can read more about in Bongaardt (2001). Bernstein (1984/1935, p. 355) stated that:
In the context of motor control, a degree of freedom can be described as the smallest number of coordinates required to describe the state of a system. For example, the proximal interphalangeal (PIP) joint of the 4th digit has one degree of freedom, as it has one axis of movement, around which it is ‘free’ to move (i.e. flexion/extension). Therefore, just one coordinate (e.g. 45° degrees flexion from the anatomical position) is sufficient to describe the position of this system. The knee joint has two degrees of freedom, as it has two axes, (being a pivotal hinge joint, it permits flexion and extension as well as a slight medial and lateral rotation), while the hip joint has three – and so on. As each joint has a number of degrees of freedom, which can be configured in different ways with other joints to undertake an activity, a vast number of options are created. In fact, it can be shown that the number of options is in principle infinite (Latash 2008). And so far, we have only referred to position, but we should also include velocity to describe the state of a system! The question is how all these options can be coordinated, and not just in any haphazard way, but in a way that is effective, efficient and reproducible to such an extent that we can recognise people’s gait, voice or hand writing? Taken together, ‘coordination’ is quite a considerable engineering problem, which is known as ‘the degrees of-freedom problem’, ‘the Bernstein problem’ or ‘the problem of motor redundancy’ (Latash, 2008, Turvey 1990).
According to Bernstein, coordination involves the control of redundant degrees of freedom, turning it into a system that can be controlled. Clinical application box 7.1 may help to clarify what is meant by this idea.
Clinical application box 7.1 Mastering degrees of freedom
The neurological condition of cerebral palsy (CP) was introduced in Chapter 6. CP may have wide-ranging effects on a child’s development, and include motor, psychological and social difficulties. One particular motor impairment that may result from CP is ataxia, whereby the coordination of movement is disorganised. Children with CP may be unable to control their sitting position and have profound difficulty with eye–hand coordination. Lacking an adequate base of support, the control of head, eye and upper limb movement is even further compromised. Butler (1998) and Major et al. (2001) devised an intervention known as ‘targeted rehabilitation’, utilising a frame to immobilise different body segments (e.g. ankles, knees, hips, pelvis and/ or trunk) while leaving others free to move – depending on the child’s level of coordination. The reasoning is that, due to the cerebral palsy, children are unable to intrinsically control the numerous degrees of freedom associated with an upright position. Targeted rehabilitation utilises a supportive device in which the child can sit or stand, using supports in specific locations. Control of head position in space is the first target, as this comprises the important sensory systems of vision, balance and hearing that play a key role in posture and movement. Once this has been achieved, support will be adjusted downward, leaving just one or two joints free above the support. In this way, targeted rehabilitation systematically reduces the number of degrees of freedom by imposing external constraints on various body segments and reduces the complexity of the motor control problem. Using this method, positive findings were reported in case studies, in terms of achieving sitting balance (Butler 1998) and gait (Farmer et al. 1999).
In case study 7.1 on Parkinson’s disease (PD) at the start of this chapter, the situation is quite the opposite from the case study on ataxic cerebral palsy below; PD induces rigidity, which pathologically reduces the number of degrees of freedom available below what may be required for normal functioning. Interestingly, there is a much higher incidence of falls in PD compared with age-matched controls (Bloem et al. 2001), rising even further in studies with longer follow-up times. Could the degree-of-freedom issue provide an explanation for the high incidence of falls in PD?
Controlling degrees of freedom: modelling motor control
Before the degree-of-freedom problem had been identified in the Western world, an influential theory known as the reflex-hierarchical theory of motor control was widely accepted. This model integrates Sherrington’s work on reflexes (see Chapter 6), with a model proposed by Hughlings Jackson, according to which the brain comprises a hierarchy of levels, each higher one capable of generating more sophisticated behaviours. For example, compare the stereotypical monosynaptic stretch reflex that operates at spinal level with a voluntary complex action such as drawing, initiated from the motor cortex. This model, in which fairly autonomous reflexes were incorporated into a hierarchical control structure, had a profound influence on ideas about motor control, child development and rehabilitation. For example, early work by Bobath (1990) who revolutionalised rehabilitation for people with neurological conditions, initially focused primarily on normalising reflexes and postural tone as a prerequisite for normal movement. With the benefit of hindsight, it would have been surprising if this approach had been effective in terms of improving functional activity, as we now understand that reflexes are only one part of the complex interaction between the various body systems, environmental constraints and task requirements that play a role in functional activity – and this has been incorporated in a more modern interpretation of Bobath (Raine 2009). A full discussion of the reflex-hierarchical theory of motor control, and implications for clinical practice are beyond the scope of this book and readers are referred to Gordon (2000) and Kamm et al. (1990).
More contemporary motor control theories can be categorised by dividing them into two broad frameworks: ‘motor systems theories’ and ‘action systems theories’ (Meijer and Roth 1988):
• ‘Motor systems’ theories (also known as the motor programming or information processing approaches), comprise the more traditional approach to motor control. Stemming from the background of cognitive psychology, this approach proposes that movement is controlled by programme that have been stored in memory. These theories concentrate on what information is necessary to control movement, and the role of cognition in this process.
• ‘Action systems’ theories are in direct contrast with the former as they question – if not reject – the role of cognition in motor control. They argue instead that the information required to control movement is directly available to the organism (the ecological approach to perception, or direct perception approach) or that movement is subject to laws of physics and thereby largely self-organising (the dynamical systems or natural-physical approach).
Motor control: a motor programming approach
A hierarchical model of motor control: an introduction
We will start our discussion with the motor programming approach to motor control. For a potted history of the science of motor control, the reader is referred to a particularly illuminating account by Meijer (2001).
The motor programming approach to motor control represents a group of methodologies including those that study the role of information processing in motor control, modelling the nervous system as a virtual neural network through computer simulations, or signal processing in the actual nervous system. Central to this approach is the concept of information processing. The first person to propose that the human brain can be compared to a computer, as they both receive and process sensory information and produce output, was Craik in 1948 (in Summers 2004). This metaphor was hugely influential in psychology and inspired information processing theories of a wide range of human behaviours, including motor control and skill acquisition.
Among the first psychological theories about motor behaviour based on this metaphor, those postulated by Adams (1971) and his student Schmidt (1975) have been and continue to be particularly influential (Summers 2004). Both came from a background of physical education and were interested in increasing their understanding of human motor control to enable them to design effective training programmes. A historical overview of the development of this approach to motor control and learning can be found in Schmidt (1988) and Summers (2004).
The model of motor control, currently proposed by Schmidt and Wrisberg (2008), is outlined in Figure 7.1. This model presents the subsequent stages of information processing, involved in generating motor output. Let’s go through this model by using the example from the PD case study: stepping onto a stepping stone.
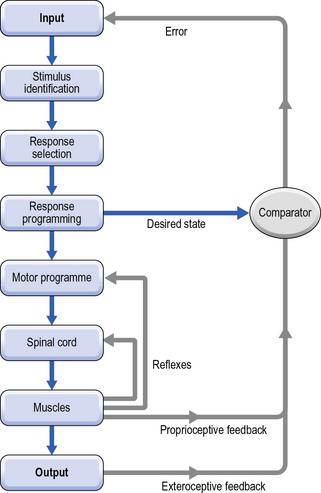
Fig. 7.1 • A motor programming model of motor control,
according to Schmidt and Wrisberg (2008), with permission from Human Kinetics.
When completed, the desired state is fed forward to the Comparator, to update it on the intended action. Having a plan of the intended action will later enable the comparator to match the intended with the actual action and generate an error signal should there be any discrepancy between the two. As we will see in Chapter 8, this stage is essential for skill acquisition.
Once compiled, the Motor Programme is then fired off via the final common path to the spinal cord, alpha motor neurones and the motor end plates, activating muscles – with motor output as a result. Following Chapter 6, this part of the process will now be familiar to you. Although the motor programme is finally realised through muscle activations, the controlling parameters used by the central nervous system are not necessarily expressed in terms of muscle parameters initially, as will be explained later (Section 3: Response programming: invariants and parameters).
Several forms of feedback ensue from this process: muscle reflexes are likely to be evoked; monosynaptic stretch reflexes (M1), longer duration functional stretch reflexes (M2) and voluntary reactions (M3) are all elicited to regulate posture and balance during the intended action (see Chapter 6). Proprioceptive feedback from muscle spindles, tendons and ligaments is also generated, providing information about posture and movement. Finally, exteroceptive feedback emerges, which is feedback about the environment (e.g. vision tells you that you have reached/missed your target). This actual output is then fed into the comparator which, as explained above, compares the actual state with the intended state. If the two signals match, this indicates that all has gone to plan, and no error signal is generated. However, if there is a difference between the two, an error signal is fed back into the system as Input. If there is sufficient time for the action (e.g. self-paced stepping), this information is used by the organism to re-adjust its action as this unfolds. Going round the entire loop (i.e. from input, via exteroceptive feedback, and onto further input) takes around 300 ms. However, as we shall see, some actions (e.g. jumping) are too fast and feedback may not be processed in time for the current action. This means that you need to store the information and wait until the next try.
Open and closed loop control
Following this outline, we shall now examine the model in more detail. As Figure 7.1 suggests, the model comprises two components; an Open Loop (blue) and a Closed Loop component (grey). The Closed Loop component was proposed by Adams in the 1970s (Adams 1971). This component represents a full loop through all stages of the model, utilising feedback during and following the movement. Provided that an action is sufficiently slow, exteroceptive and proprioceptive feedback can be utilised to ‘steer’ the activity as required. The minimum time needed for a response to this visual and proprioceptive feedback is estimated at about 100 ms (Jeannerod 1988). For example, handwriting tends to be a slow and deliberate activity. The position of the pen in relation to the paper is carefully monitored on-line and adjustments can be made as the activity proceeds. This way, errors that are too small to be detected consciously, can be corrected. However, closed loop control on its own does not suffice for ballistic movements (e.g. kicking, jumping, punching) or ballistic components of a movement (e.g. initial phase of reach-to-grasp) that are faster than this.
Together, by having a higher order Open Loop with a Closed Loop mode of motor control nested within, Schmidt’s hierarchical model (Schmidt and Wrisberg, 2008) is able to describe how fast, as well as slow movements are controlled, and explain how errors can be corrected – depending on the amount of time available.
In summary, a motor programme is a template for motor control; a set of motor commands that prescribes the essential characteristics of a skilled action (Schmidt and Wrisberg 2008).
The generalised motor programme
It is important to highlight at this point that, rather than each movement having its own dedicated motor programme, Schmidt proposed that classes of action may be discerned (e.g. walking, reaching, rising from sit to stand). Each class of action is purported to have its own kinematic ‘signature’, or pattern, and all movements within the same class of action are controlled by the same motor programme, known as a generalised motor programme (GMP). So for example, the GMP for reaching comprises certain key components, as Research box 7.1 below explains.
Research box 7.1 Example: Motor programme for reach-to-grasp
In healthy subjects, for a given reach-to-grasp movement the hand follows a characteristic path and trajectory as it moves towards an object, described as the ‘transport’ component (change over time of the position of the wrist (Jeannerod 1984)) and the hand opens and closes on the object, the ‘grasp’ component (change over time of the distance between the index finger and thumb (Jeannerod 1984)). Neurophysiological evidence supports separate but interdependent visuomotor control channels for these two components (Ungerleider and Mishkin, 1982, Goodale and Milner 1992). The velocity profile of the hand is typically asymmetric, with the peak velocity achieved within 50% of movement duration. The time to PV is often described as ‘ballistic’ meaning it is assumed to be driven by force commands that have been planned and activated prior to the beginning of the movement (open loop control) (Nagasaki 1989). The time after PV is thought to be controlled in a feedback manner whereby part by visual or proprioceptive information made available to the central nervous system after the movement begins is used to correct the movement according to task goals and environmental demands. The time after PV is also described as the ‘deceleration’ phase, while the hand slows in readiness to grasp the object.
When the task requirement for the grasp is changed by making an object smaller, or by requiring a more accurate task to be performed with then object, peak velocity occurs earlier, resulting in a longer deceleration phase (Marteniuk et al. 1987), allowing time to process the various sources of sensory feedback.
When the task requirement for the transport is changed by increasing the speed of the reaching movement, the maximum amount of hand opening increases (Wing et al. 1986).