Positron Emission Tomography in Ischemic and Hemorrhagic Stroke
Pearls
Positron emission tomography (PET) measurements of hemodynamic parameters, including cerebral blood flow and oxygen extraction fraction, are not Food and Drug Administration (FDA)-approved for diagnostic use and are presently used for research purposes only. PET studies have yielded important knowledge regarding human cerebrovascular disease, including the following pearls.
The best indicator of viable penumbral tissue in acute ischemic stroke is preserved oxygen metabolism. Flow parameters are not specific for whether tissue is alive or dead.
Autoregulation is intact in tissue around an acute ischemic infarction.
There is no evidence that intracranial hemorrhage causes ischemic changes in adjacent brain.
Autoregulation is frequently impaired in patients with aneurysmal-subarachnoid hemorrhage (SAH)-induced vasospasm.
Positron emission tomography (PET) remains an important research tool for in vivo investigations of human cerebrovascular pathophysiology. PET imaging provides regional, quantitative measurements of important parameters such as cerebral blood flow (CBF) and oxygen metabolism, as well as molecular imaging using specific physiologic and pathologic chemical compounds (molecular imaging). The measurements are also useful in the assessment of therapeutic interventions, such as red cell transfusion in cerebral vasospasm, for example, on different physiologic parameters. The ability to measure oxygen metabolism, in addition to CBF, facilitates the comprehensive assessment of the impact of therapeutic interventions on the ischemic vulnerability of brain tissue. Many of these applications are unique to PET imaging. This chapter reviews the knowledge gained from PET studies regarding the pathophysiology and treatment of acute ischemic and hemorrhagic stroke, as well as chronic oligemia from arterial occlusive disease. The first section is a review of the basic principles and important limitations of PET. The second section is a review of normal cerebral hemodynamics and metabolism. The third section is a discussion of the responses of the brain and its vasculature to reductions in perfusion pressure, primarily autoregulatory vasodilation and increased oxygen extraction fraction. The remaining sections briefly review the contributions of PET studies to our understanding of pathophysiology and treatment in the settings of chronic arterial occlusive disease, acute ischemic stroke, acute intracerebral hemorrhage, and subarachnoid hemorrhage.
♦ Positron Emission Tomography Imaging Physics
Positron emission tomography imaging requires two or three components: a positron-emitting isotope (radiotracer), a tomographic imaging system to detect the location and to measure the quantity of radiation, and often a mathematical model relating the physiologic process under study to the detected radiation.1 For example, the method used in our laboratory for the measurement of cerebral blood flow uses a bolus injection of O–15 labeled water (H2 15O, the radiotracer).1 The PET camera system records the location and number of counts during the circulation of the water through the brain. Finally, the tomographic PET images of raw counts are converted into maps of regional quantitative CBF using computer algorithms. This processing requires measurement of arterial blood counts and incorporates models and assumptions regarding the transit of water through the cerebral circulation.
Radiotracers are radioactive molecules administered in such small quantities that they do not affect the physiologic process under study. PET radiotracers decay by positron emission and may be separated into two broad categories: normal biologic molecules, such as 15O-labeled water, or nonbiologic elements attached to organic molecules as radiolabels, such as 18F-labeled deoxyglucose.
Positron emission tomography imaging detection systems use the phenomenon of annihilation radiation both to localize and to measure physiologic processes in the brain. In the body, the positron (a positively charged electron emitted by the radionuclide) travels up to a few millimeters before encountering an electron. This encounter results in the annihilation of both the positron and electron and the consequent generation of two gamma photons of equal energy. These two photons are emitted in characteristic 180-degree opposite directions. A pair of detectors positioned on either side of the source of the annihilation photons detects them simultaneously. This allows localization of the point source of the radiation.
The most important limitations of PET imaging of physiologic processes relate to the phenomenon of full-width, half-maximum (FWHM) and a related phenomenon of partialvolume averaging. Detected radiation is observed over a larger area than the actual source. The spread or distribution of activity is approximately gaussian for a point source of radiation, with the maximum located at the original point. The FWHM describes the degree of smearing of radioactivity in a reconstructed image. The ability of a PET scanner to discriminate between two small adjacent structures or accurately measure the activity in a small region depends on the FWHM of the system as well as on the amount and distribution of activity within the region of interest and the surrounding areas. Because of the smearing or redistribution of detected radioactivity, any given region in the reconstructed image does not contain all the activity actually within the region. Some of the activity spills over into adjacent areas. This phenomenon is known as the partial-volume effect. An important consequence of this principle is that PET always measures a gradual change in activity where an abrupt change actually exists, such as in an infarct, hemorrhage, or at the border of different structures such as the brain and cerebrospinal fluid (CSF) or gray and white matter.2
Finally, the externally measured tissue concentration of the positron-emitting radiotracer (PET counts) is quantitatively related to the physiologic variable under study by a mathematical model. The PET scanner measures the total counts in a volume of tissue over time. The model then calculates how that measured activity reflects the physiologic parameter under study. These calculations account for several factors related to the tracer biomechanics and metabolism. These factors include the mode of tracer delivery to the tissue, the distribution and metabolism of the tracer within the tissue, the egress of the tracer and metabolites from the tissue, the recirculation of both the tracer and its labeled metabolites, and the amount of tracer and metabolites remaining in the blood.
♦ Normal Cerebral Hemodynamics and Metabolism
A brief introduction and definition of the common physiologic parameters measured with PET is useful prior to the discussion of normal hemodynamics and metabolism. Cerebral blood flow (CBF) is the volume of blood delivered to a defined mass of tissue per unit time, generally in milliliters of blood per 100 g of brain per minute (mL/(100 g · min)) ( Fig. 9.1 ). 15O-labeled water is the most commonly used tracer for measurements of CBF and the method used in our laboratory.1 Other methods are in common use as well, including O–15 labeled butanol.3
Cerebral blood volume (CBV) is the volume of blood within a given mass of tissue and is expressed as milliliters of blood per 100 g of brain tissue. Regional CBV measurements may serve as an indicator of the degree of cerebrovascular vasodilatation, as discussed below. CBV can be measured by PET with either trace amounts of 15O-labeled carbon monoxide or 11CO1 ( Fig. 9.1 ). Both carbon monoxide tracers label the red blood cells. Blood volume is then calculated using a correction factor for the difference between peripheral vessel and cerebral vessel hematocrit. Mean transit time (MTT) is usually calculated as the ratio of CBV/CBF. By the central volume theorem, this ratio yields mean transit time, the hypothetical mean time for a particle to pass through the cerebral circulation. Increased MTT is used as an indicator of autoregulatory vasodilation. Some PET groups have advocated the use of the inverse of this ratio instead.4
Oxygen extraction fraction (OEF) is the proportion of oxygen delivered that is extracted by tissue for metabolism. In the brain OEF normally varies between 0.25 and 0.5, with values over 0.5 signifying increased extraction. It is measured in our laboratory by an O15O inhalation scan and independent measurements of CBF and CBV1 ( Fig. 9.1 ). The CBF accounts for the amount of oxygen delivered to the brain. The CBV corrects for oxygen in the blood that is not extracted. An alternative count based method uses the ratio of the counts after an O15O inhalation scan to the counts from an O15O water scan, without CBV correction.5 Other similar methods are also in common use. Cerebral metabolic rate of oxygen (CMRO2) is the amount of oxygen consumed by tissue metabolism, measured in milliliters of oxygen per 100 g of brain tissue per minute1 ( Fig. 9.1 ). CMRO2 is equal to the CBF multiplied by OEF and the CaO2 (delivery of oxygen times the fraction extracted times the amount of available oxygen).
Molecular imaging studies in ischemic and hemorrhagic stroke patients have used several different labeled substances,6 including 11-C-labeled FK506 (tacrolimus) copper-60, 11C-flumazenil (FMZ), 18F-fluoromisonidazole (18F-FMISO), and a host of other labeled neurotransmitters. Glucose metabolism (CMRGlu) is most frequently measured using the glucose analogue 18F-fluorodeoxyglucose (FDG). CMRGlu measurements are limited in pathologic conditions such as ischemia, however, because the ratio of tissue uptake of glucose and its analogue, DG, varies with the severity of ischemia. Glucose metabolism can be measured directly with emia. Glucose metabolism can be measured directly with 11C-D-glucose.7
Whole-brain mean CBF of the adult human brain is approximately 50 mL per 100 g per minute. Functional activation increases local or regional CBF, but global CBF generally remains unchanged. CBF for any brain region is determined by the ratio of cerebral perfusion pressure (CPP) and cerebral vascular resistance (CVR) in that region. Cerebral perfusion pressure is the difference between the arterial pressure forcing blood into the cerebral circulation and the intracranial pressure or the pressure in the venous system. The venous pressure is negligible under most conditions, so the CPP is generally equal to the systemic arterial pressure. Several different pathologic processes result in reduced perfusion pressure secondary to venous disease, however. These include venous sinus thrombosis, dural arteriovenous fistulas, and possibly other conditions such as jugular foraminal narrowing. The venous pressure also increases with increased intracranial pressure.

Under normal conditions any change in regional CBF (rCBF) must be caused by a change in regional CVR. Vascular resistance is mediated by alterations in the diameter of small arteries or arterioles. In the resting brain with normal CPP, CBF is also closely matched to the metabolic rate of the tissue. Regions with higher metabolic rates have higher levels of CBF. For example, gray matter has a higher CBF than white matter. Although there is wide variation in levels of flow and metabolism, the ratio between rCBF and metabolism is nearly constant in all areas of the brain. Consequently, the maps of OEF and glucose extraction (not metabolism) from the blood show little regional variation.8 One exception to this is seen with physiologic activation, where blood flow increases well beyond the metabolic needs of the tissue. This leads to a relative decrease of OEF and a reduction in local venous deoxyhemoglobin.9 This phenomenon is the basis for the use of magnetic resonance imaging (MRI) as a means to map brain function.
♦ Responses to Reductions in Cerebral Perfusion Pressure: Oligemia and Ischemia
An arterial stenosis or occlusion may cause a reduction in perfusion pressure if collateral sources of flow are not adequate.10 The presence of arterial stenosis or occlusion does not equate with hemodynamic impairment: up to 50% of patients with complete carotid artery occlusion and prior ischemic symptoms have no evidence of reduced CPP.11 The adequacy of collateral sources of flow determines whether an occlusive lesion will cause a reduction in perfusion pressure. When perfusion pressure falls owing to an occlusive lesion and an inadequate collateral system, the brain and its vasculature maintain the normal delivery of oxygen and glucose through two mechanisms: autoregulatory vasodilation and increased oxygen extraction fraction.12 The presence of these mechanisms has been extensively studied, primarily in animal models employing acute reductions in perfusion pressure. The extent to which these models are applicable to humans with chronic regional reductions in perfusion pressure is not completely known. Autoregulatory vasodilation and increased OEF may also occur in response to reduced CPP owing to increases in venous back pressure.13
Changes in perfusion pressure have little effect on CBF over a wide range of pressure owing to vascular autoregulation. Increases in mean arterial pressure produce vasoconstriction of the pial arterioles, serving to increase vascular resistance and maintain CBF at a constant level.14 Conversely, when the pressure falls, reflex vasodilation maintains CBF at near-normal levels.15 Two measurable parameters that indicate autoregulatory vasodilation are increases in MTT and CBV ( Fig. 9.2 ). Despite vasodilation, there is some slight reduction in CBF through the autoregulatory range as perfusion falls, leading to a slight increase in oxygen extraction to compensate for the reduced delivery of oxygen.12

At some point the capacity for autoregulatory vasodilation can be exceeded. The threshold value for autoregulatory failure is variable between patients and can be shifted higher or lower by prior ischemic injury or long-standing hypertension. Beyond this point, CBF falls linearly as a function of pressure. Direct measurements of arteriovenous oxygen differences (CaO2 × OEF) using jugular venous oximetry have demonstrated the brain’s capacity to increase oxygen extraction (OEF) and maintain normal cerebral oxygen metabolism (CMRO2) in circumstances where oxygen delivery diminishes due to decreasing CBF16 ( Figs. 9.1 and 9.2 ). The precise mechanism by which OEF increases is not completely understood. Oxygen passively diffuses from the blood to the tissue. The best current hypothesis is that more of the oxygen that diffuses into the tissue is used for oxidative metabolism, thus reducing the amount of oxygen available to diffuse back to the capillaries.17
If the perfusion pressure of the brain continues to fall beyond the capacity for increases in OEF to compensate for the reduced delivery of oxygen, oxygen extraction becomes insufficient to meet the energy requirements of the brain.18 CMRO2 begins to fall and neurologic dysfunction occurs. This may be reversible if oxygen delivery is rapidly restored. Persistent or further declines in flow can lead to permanent tissue damage, depending on the duration and degree of ischemia.19 Below approximately 20 mL per 100 g per minute, normal brain electrical activity ceases and neurologic symptoms may appear. The energy supply becomes insufficient owing to the inadequate supply of oxygen, preventing normal aerobic glycolysis. The high-energy phosphate stores of adenosine triphosphate (ATP) and phosphocreatine (PCr) become depleted. Anaerobic metabolism of the small amount of glucose remaining in the intracellular stores or from the diminished blood flow leads to a lactic acidosis. Once CBF has fallen to 10 to 12 mL per 100 g per minute, the integrity of cell membranes is lost and intracellular K+ leaks out of the cells whereas extracellular Ca+ leaks in. Cell death ultimately follows unless reperfusion occurs quickly.
Once tissue damage has occurred, the normal mechanisms of cerebrovascular control may no longer operate.20 Therefore, in some patients who have had transient ischemic attacks (TIA) or mild ischemic strokes with subsequent recanalization, autoregulation or the normal cerebrovascular response to PaCO2 may be abnormal for up to several weeks.21 Over time, flow falls to match the metabolic needs of the tissue, and autoregulatory capacity is regained. Following reperfusion, the biochemical and ionic abnormalities resolve to a degree dependent on the severity of the initial ischemic insult. The acidosis of anaerobic glycolysis may be replaced by alkalosis.
Chronic oligemia may lead to other compensatory mechanisms, in addition to autoregulatory vasodilation and increased OEF. These include possible reversible metabolic down regulation, accompanied by a reversible cognitive impairment.22 This phenomenon remains an unproven hypothesis and is being evaluated in ongoing trials.
♦ Positron Emission Tomography PET Studies in Chronic Arterial Occlusive Disease (Oligemia)
The identification of compensatory responses to reduced perfusion pressure, or hemodynamic impairment as it is frequently called, may play an important role in medical decision making in several subacute or chronic arterial occlusive disorders. These conditions include atherosclerotic carotid occlusion, arterial dissection, moyamoya disease, and possibly asymptomatic atherosclerotic carotid stenosis. PET and other hemodynamic studies in these patient populations have been aimed primarily at establishing if the presence of these compensatory mechanisms is associated with future stroke risk (natural history studies), and if particular medical or surgical interventions can improve cerebral hemodynamics (i.e., using imaging as a secondary end point). Pivotal intervention studies of efficacy based on hemodynamic criteria have also been done. This section reviews PET methods for identification of autoregulatory vasodilation and increased OEF, and reviews clinical studies in different patient populations.
Identification of Compensatory Reponses to Reduced Perfusion Pressure with Positron Emission Tomography
As discussed above, the hemodynamic effect of an arterial stenosis or occlusion depends on the adequacy of collateral circulation as well as the degree of stenosis. An occluded carotid artery, for example, often has no measurable effect on the distal CPP because the collateral flow through the circle of Willis is adequate. Many imaging techniques, such as arteriography, MRI, computed tomography angiography, and Doppler ultrasound, can identify the presence of these collaterals. These tools show us the highways for blood flow, but not the traffic on them.
It is important to recognize that a single measurement of flow is meaningless when investigating the effects of an arterial lesion. Normal values of CBF do not exclude the presence of autoregulatory vasodilation, and reduced CBF may be present with normal perfusion pressure. This second situation may occur with prior stroke in the region of interest or in a remote area. Prior lacunar stroke in the basal ganglia may lead to profound reduction in metabolic demand of the overlying cortex and secondary reduction in flow. This phenomenon has been termed diaschisis ( Fig. 9.3 ).
Three basic PET strategies for defining the degree of hemodynamic compromise caused by arterial occlusive disease have emerged, based on the known compensatory responses of the brain to reduction in CPP as discussed above. Two approaches are used to identify autoregulatory vasodilation and the third identifies increased OEF. The first method relies on resting measurements of CBV and CBF. When CPP is reduced, autoregulatory vasodilatation causes an increase in CBV. The CBV/CBF ratio (MTT) increases. The second strategy employs paired measurements of rCBF at rest and during some form of vasodilatory stimulus, such as with acetazolamide (Diamox) or CO2 inhalation. Reduction of the normal increase in CBF seen in response to these stimuli is taken as evidence of preexisting autoregulatory vasodilatation, which would mute such an increase.

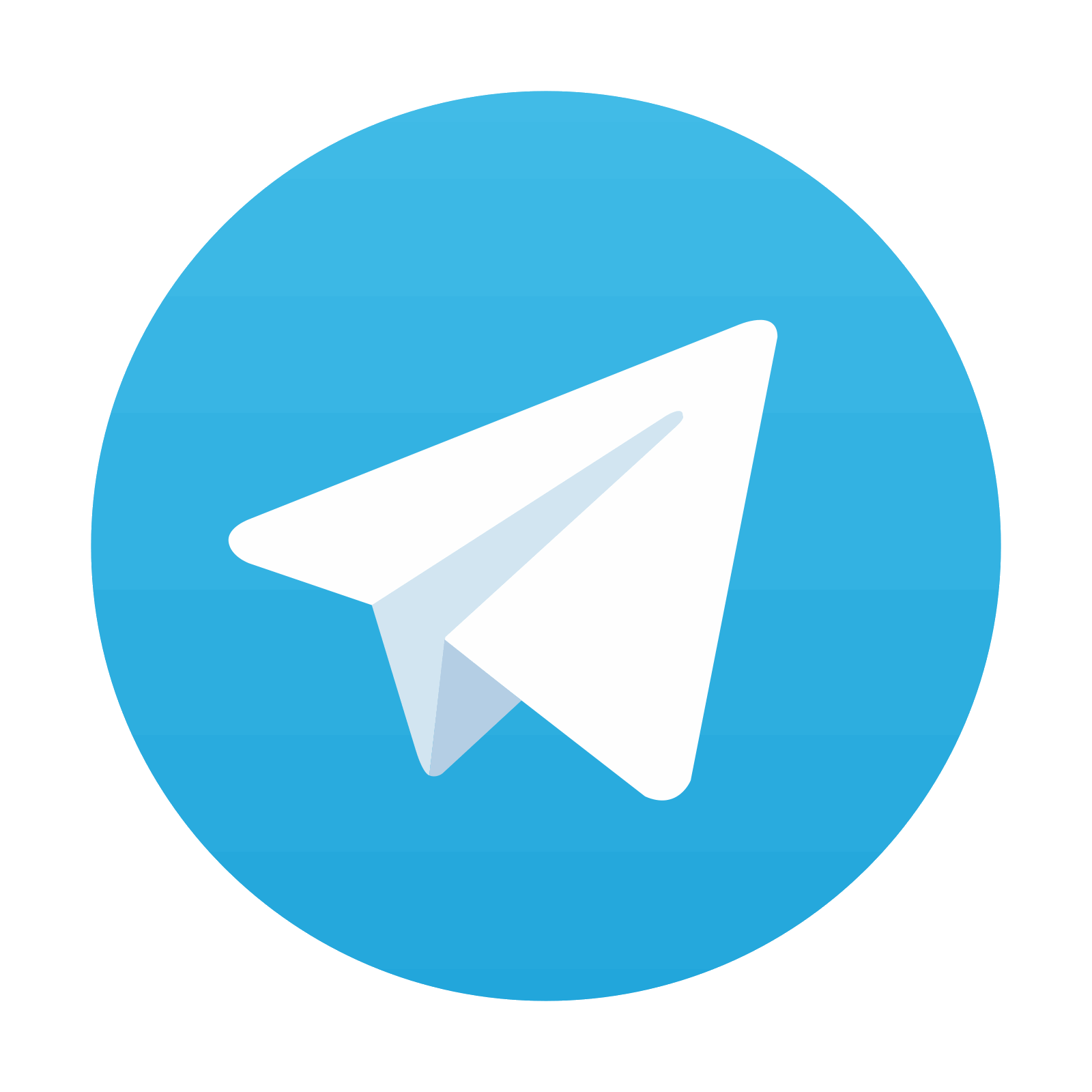
Stay updated, free articles. Join our Telegram channel
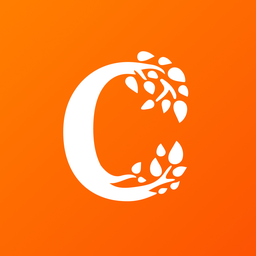
Full access? Get Clinical Tree
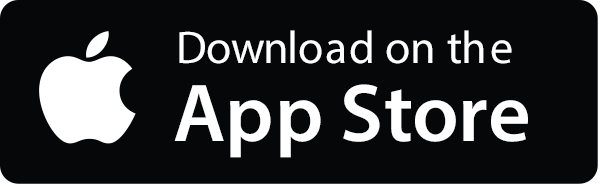
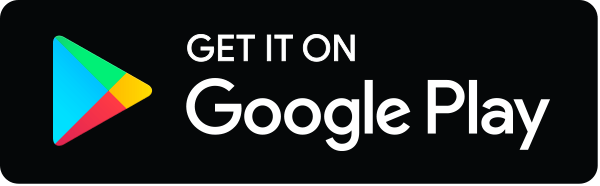
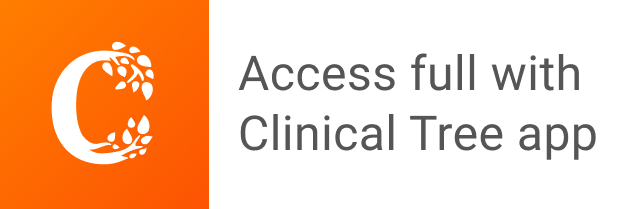