Postnatal Trauma and Injuries by Physical Agents
John H. Menkes
Richard G. Ellenbogen
CRANIOCEREBRAL TRAUMA
In the Western world, accidents constitute the major cause of death of children between the ages of 5 and 19 years (Table 9.1) (1). From 1951 to 1971, the number of children with head injuries admitted to hospitals in Newcastle-upon-Tyne, United Kingdom, increased sixfold, reaching 13.9% of all admissions to pediatric wards (2). This increase probably was partially caused by a change in policy such that more apparently minor injuries were admitted for observation. In the United States, the situation is similar; cranial and major facial injuries are responsible for 3.6% of hospital admissions and 3.3% of days spent in the hospital, and they are the most common neurologic conditions requiring hospital admission for patients younger than 19 years of age (3). Head injury is now the most common cause of death and disability of children in the United States, causing death in approximately 7,000 children each year (4). It is also the cause of significant cognitive and motor-sensory dysfunction in the pediatric population, with an estimated economic burden approximating $10 billion a year in the United States alone (5).
A high proportion of head injuries cause death at the site of the accident or on the way to the hospital, so admission figures reflect only part of the incidence. In the San Diego Prospective Survey, as many as 85% of fatalities occurred at these times (6). Since the 1980s, child abuse has been recognized increasingly. The incidence of reported cases rose from 10.1 in 1,000 children in 1976 to 39 in 1,000 children in 1990 (7), with one of the more recent estimates of the incidence of fatal abuse in children younger than 4 years of age being at least 10 in 100,000 (8). As the reported frequency of maltreatment has risen, it has become an important cause of head injury. In one series, in infants younger than age 1 year, 36% of all head injuries and 95% of injuries resulting in intracranial hemorrhage or other major cerebral complications were caused by child abuse (9). In older children, the proportion of injury from abuse is lower, but still significant. In addition, children who were hospitalized as a consequence of child abuse remained in the hospital more than twice as long as other hospitalized children (10).
Although no accurate data exist, cranial trauma commonly occurs in childhood sports. Brain concussion occurs in an estimated 19 in 100 American football players per year. Bicycling is probably the second-most-common sport leading to head injury. In an Australian series published in 1987, bicycling was responsible for more than 20% of all head injuries in children (11). The remarkable effectiveness of bicycle helmets in preventing head injuries in children has been attested to in several studies (12,13).
This chapter presents only postnatal injuries; perinatal injuries are described in Chapter 6. This chapter discusses the diagnosis and nonsurgical management of head injuries and considers their pathophysiology and pathology based on the severity of the craniocerebral trauma and the complications and sequelae of head injuries.
Dynamics and Pathophysiology
The adverse effects of head injury are primary and secondary. The primary effects are the result of physical forces, mainly acceleration and deceleration, that act on the brain through shear-strain deformation (a change in shape without a change in volume) and through compression-rarefaction strain (a change in volume without a change in shape). The brain is injured through these two mechanisms acting alone, in combination, or in succession (14,15,16). Secondary effects result from the various processes that complicate the injury.
Shear-Strain Deformation
Shear strain occurs when two layers slide on each other, moving in parallel in opposite directions (16). The injury from shearing or tearing is responsible for most lesions, especially in an infant or a young child, whose skull is more easily deformed than an adult’s. Deformation
absorbs much of the energy of impact, reducing the adverse effects of acceleration and deceleration but adding to the risk of tearing of blood vessels. Because of its elasticity and ability to undergo a greater degree of deformation, the skull of an infant absorbs the energy of the physical impact and protects the brain better than the skull of an older person.
absorbs much of the energy of impact, reducing the adverse effects of acceleration and deceleration but adding to the risk of tearing of blood vessels. Because of its elasticity and ability to undergo a greater degree of deformation, the skull of an infant absorbs the energy of the physical impact and protects the brain better than the skull of an older person.
TABLE 9.1 Causes of Death in Children in 1986 | ||||||||||||||||||||||||||||||||||||||||
---|---|---|---|---|---|---|---|---|---|---|---|---|---|---|---|---|---|---|---|---|---|---|---|---|---|---|---|---|---|---|---|---|---|---|---|---|---|---|---|---|
|
The relationship between stress waves and deformity depends mainly on the momentum of the head and of the object at the instant of impact. In a child with intact reflexes, a free fall probably causes more injury to the brain than an aimed object striking the head with even greater speed. Approximately 70% of shear-strain deformation skull fractures are linear and single; the rest are depressed fractures. The faster moving the object delivering the blow, the more likely it is that a depressed fracture will result; a lower-velocity impact with the same momentum tends to produce deformity and linear fracture, sometimes distant from the point of impact but at a weak part of the cranial vault where distortion can occur. In addition, the size of the object that strikes the skull is important. Objects smaller than 5 cm2 tend to produce a depressed fracture; larger objects tend to produce a linear fracture (16). A linear fracture is usually not important in management of the child because outcome of the injury and its complications are determined by damage to the intracranial contents at the moment of impact; an injury can be fatal in the absence of a fracture.
Compression-Rarefaction Strains
In an acceleration injury, in which the momentary compression is greatest, the effects of compression-rarefaction strains are usually maximal at the point of impact; at the same time, an area of low pressure or rarefaction occurs contralaterally, the site of the familiar contrecoup injury (13,14), Thus, a blow to the occiput can result in the major damage in the frontal and temporal regions. Contrecoup injuries generally occur on the undersurface and poles of the frontal and temporal lobes (16). They are relatively rare in infants and young children, presumably because skull distortion rather than pressure waves predominate (19). Contrecoup injuries are most likely to occur when the impact is to the occiput or to the side of the head (16). Distortion of the brainstem is particularly likely in deceleration injuries such as those that occur in falls; any resulting loss of function in this area is liable to have serious or fatal consequences.
Magnetic resonance imaging (MRI) has allowed a better understanding of the lesions that can result from head injury. These were grouped into primary and secondary lesions by Gentry and coworkers (20) (Table 9.2). The injuries expected from various accident mechanisms are depicted in Table 9.3.
TABLE 9.2 Classification of Traumatic Intracranial Lesions | ||
---|---|---|
|
TABLE 9.3 Expected Injury Types Associated with Accident Mechanisms in Young Children | ||||||||||
---|---|---|---|---|---|---|---|---|---|---|
|
Diffuse Axonal Injury
The term diffuse axonal injury (DAI) refers to a clinical-pathologic-radiologic entity that clinically manifests itself by loss or impairment of consciousness.
The lesion usually is not the result of a fall, except when the fall occurs from a considerable height. Instead, it results from severe angular acceleration-deceleration forces (21) and is believed to induce coma through disconnection of the cortex from the lower centers. It is responsible for severe, irreversible, and potentially fatal brain damage occurring at the moment of injury. DAI is frequently unaccompanied by skull fracture, increased intracranial pressure, or cerebral contusion (21).
As viewed microscopically, the hallmark of DAI is the presence of axonal retraction balls identified by silver stains or, as in more recent studies, immunohistochemical staining for beta-amyloid precursor protein (16,22). Postmortem examinations have demonstrated axonal lesions in the inferior portion of the corpus callosum and the dorsolateral quadrant of the rostral brainstem, notably in the region of the superior cerebellar peduncles, and throughout the cerebral hemisphere and cerebellum (23,24,25). Damage to the superior cerebellar peduncles, which are particularly vulnerable to rotational injuries, is responsible for the ataxia commonly observed after major head injuries (26).
The microscopic picture of axonal lesions was first described by Strich (27), who proposed that the shearing forces sustained during injury cause stretching of axons, which might be sufficient to prevent them from functioning. The more subtle changes that precede this final state have been studied in animals by electron microscopy and through the use of immunocytochemical labeling techniques. Although the exact time course for humans has not been determined, in animals an alteration in the structure of the nodes of Ranvier occurs within 15 minutes of the injury (28). This is followed some 12 to 24 hours later by an interruption of antegrade and retrograde axonal transport, the loss of microtubules, and the gradual development of axonal swelling. When axonal swelling exceeds a certain critical level, effective transection of the axon occurs, although without evident tearing or damage to adjacent blood vessels. DAI is frequently accompanied by evidence of neuronal injury (29).
It is unclear how mechanical forces induce the instantaneous perturbation of the cell membrane that in turn initiates these axonal changes. Wolf and coworkers proposed that traumatic deformation of axons induces abnormal sodium influx through mechanically sensitive Na+ channels. This influx subsequently triggers an increase in intraaxonal calcium through the opening of the voltage-gated calcium channel (30). This can result in activation of calmodulin and an increase of extracellular potassium at the damaged node (31). Intracellular calcium, in turn, increases the activity of proteolytic enzymes that disrupt axonal cytoarchitecture. Over time, the swollen axons either degenerate or undergo regenerative changes, as shown by sprouting and growth cones (32).
On MRI, axonal damage is best visualized by diffusion tensor imaging (DTI) or high-spatial-resolution susceptibility-weighted imaging techniques (33,34). By these techniques diffuse axonal injury appears as small, oval, focal abnormalities in white matter tracts, usually adjacent to cortical gray matter, but sometimes in the splenium of the corpus callosum (20). Proton magnetic resonance spectroscopy can provide further information on the extent of axonal injury. A lowered ratio of N-acetylaspartate (NAA) to creatine is believed to be indicative of axonal injury, but there does not appear to be any elevation of tissue lactate (35).
Cortical Contusion
The second-most-common type of brain injury visualized by MRI of patients with severe head trauma is cortical contusions, which tend to be multiple and represent bruises on the surface of the brain. As verified by both imaging and pathologic studies, points of predilection for contusions are the crests of gyri on the orbital surfaces of the frontal lobes and the inferolateral aspect of the temporal lobes. Contusions consist mainly of petechial hemorrhages in the superficial cortical layers occurring at the site of impact (coup injuries) or at contrecoup areas (Fig. 9.1). Impact on the forehead or vertex can send the initial pressure wave caudally, leading to downward displacement of the brain toward and into the tentorial opening. This
displacement can result in contusions of the hippocampal gyrus, particularly the uncus, the basal ganglia, and the upper part of the brainstem (36). The severity of brain damage caused by contusion depends on the extent of vascular injury. Damage tends to be less common in infants and small children than in older children or adults subjected to comparable trauma. Instead, contusions in infants consist of slitlike tears in the cerebral white matter of the frontal and temporal lobes (16,37). One of the major clinical issues in the management of contusions is their tendency to increase in size, coalesce, or cause a mass effect, especially after the first day following injury. Therefore, a follow-up imaging study is often useful.
displacement can result in contusions of the hippocampal gyrus, particularly the uncus, the basal ganglia, and the upper part of the brainstem (36). The severity of brain damage caused by contusion depends on the extent of vascular injury. Damage tends to be less common in infants and small children than in older children or adults subjected to comparable trauma. Instead, contusions in infants consist of slitlike tears in the cerebral white matter of the frontal and temporal lobes (16,37). One of the major clinical issues in the management of contusions is their tendency to increase in size, coalesce, or cause a mass effect, especially after the first day following injury. Therefore, a follow-up imaging study is often useful.
Cerebral Laceration
Cerebral lacerations are usually the result of damage from penetrating wounds or depressed skull fractures, but they can occur without fracture in small children, whose skulls tend to become more grossly distorted at the moment of injury. Lacerations frequently involve the frontal and temporal poles and are associated primarily with tears of the dura and tears or other injuries of the major vessels and secondarily with thromboses, hemorrhages, or focal cerebral ischemia. Tears in the white matter are seen commonly in infants after blunt trauma even without fracture (38) (Fig. 9.2). They result from the soft consistency of the poorly myelinated cerebrum and from the pliancy of the immature skull.
MRI has demonstrated that macroscopic traumatic injuries to subcortical structures such as the thalamus and to the brainstem are rare. Instead, the pathologic change that results in the clinical picture generally attributed to primary brainstem damage is diffuse axonal injury.
Concussion
The physical processes within the skull caused by trauma induce numerous changes within the brain. The most common is concussion. Although there is some disagreement with respect to the definition of concussion, the American Academy of Neurology defines it as a trauma-induced alteration in mental status with or without loss of consciousness (39). In addition, there also can be post-traumatic amnesia for the moment of injury and for a variable period before it (retrograde amnesia).
The pathogenesis of concussion is under debate. From a structural viewpoint, concussion is believed to result from minor degrees of diffuse axonal injury. With more severe concussive injuries, there is a massive release of excitatory neurotransmitters, notably glutamate (40). As a result, there is a synchronized depolarization of neuronal membranes with subsequent increase of extracellular potassium (41), resulting in a functional deafferentation of the cortex (42). Compounding the disruption of ionic homeostasis is an imbalance between energy demands and supply, a loss of cerebral autoregulation in a significant proportion of patients with minor head injuries (43),
the presence of vasospasm, and a global increase in intracranial pressure, which reduce the supply of substrates to the tissue.
the presence of vasospasm, and a global increase in intracranial pressure, which reduce the supply of substrates to the tissue.
From a clinical point of view, concussion is marked by the immediate loss of consciousness, a suppression of reflexes, a transient arrest in respiration, accompanied by bradycardia and a fall in blood pressure. Vital signs quickly stabilize, and there is a gradual return of consciousness. However, neuropsychologic testing will show deficits for as long as 15 minutes after the injury. With increasing injury, the loss of consciousness is prolonged and there is an increasing duration of post-traumatic amnesia (44). The clinical features of postconcussion syndrome are covered in another part of this chapter.
Secondary Effects of Brain Trauma
Secondary effects of trauma develop as a consequence of at least five factors: cerebrovascular dysregulation, excitotoxicity, free radical formation leading to oxidative stress, energy failure, and inflammation (Fig. 9.3; Table 9.4) (45). Cerebrovascular dysregulation, excitotoxicity, and energy failure are of primary importance in the evolution of cerebral swelling. This condition has been defined as an
increase in volume caused by an increase in brain water and sodium content. Brain swelling results from increased cerebral blood volume, cerebral edema, or a combination of the two (45).
increase in volume caused by an increase in brain water and sodium content. Brain swelling results from increased cerebral blood volume, cerebral edema, or a combination of the two (45).
TABLE 9.4 Secondary Effects of Brain Trauma | |
---|---|
|
Fishman distinguished three major categories of cerebral edema: vasogenic edema, cytotoxic or cellular edema, and interstitial edema (46). Vasogenic edema is characterized by increased permeability of brain capillary endothelial cells. This results from defects in the tight endothelial cell junctions and an increased number of pinocytic vesicles, which are responsible for the transport of macromolecules across the blood–brain barrier. Vasogenic edema fluid is extracellular and accumulates primarily in white matter because resistance to fluid flow is less in white matter than in gray matter. It occurs around brain tumors, notably glioblastomas and metastatic tumors, after cerebral infarction, and in lead encephalopathy. Cytotoxic edema is marked by swelling of all cellular elements of the brain with reduction in the volume of extracellular fluid. This last form of edema is characteristic of energy depletion such as occurs during the initial stages of cerebral hypoxia. Fishman postulated a third form of brain edema in which there is an increase in water and sodium content of periventricular white matter (46). This condition is seen in obstructive hydrocephalus.
In brain trauma, the prominence of astrocytic swelling, the integrity of the interepithelial tight junctions, and the relative paucity of protein-rich fluid suggest that edema is mainly cytotoxic (47), although massive swelling of perivascular astrocytic foot processes can compress the microvasculature and reduce tissue perfusion, inducing secondary vasogenic edema.
The importance of cytotoxic edema has been confirmed by diffusion-weighted MRI performed on experimental animals. These studies demonstrate that immediately after injury, there is predominantly vasogenic edema, which, over the next few hours to days, becomes superseded and overshadowed by cytotoxic edema (48).
A number of biochemical changes follow severe traumatic head injury (Fig. 9.3, Table 9.4). In both clinical and experimental settings, good correlation exists between the severity of traumatic brain injury and the amount of neuroexcitatory amino acids, such as glutamate and aspartate, that are released (40,49). As a result, neuronal membranes depolarize with subsequent increase of extracellular potassium (42). This is accompanied by the entry of calcium into nerve terminals and is followed by further glutamate release and potassium flux. At the same time, arachidonic acid is released, which in turn induces a cascade of reactions with the ultimate formation of free radicals (50). These changes are accompanied by increased energy demands as brain cells attempt to reinstate normal ionic membrane balance (51). With an increase in energy consumption there is a rapid decrease in adenosine triphosphate (ATP) and an increase in lactic acid (52). These biochemical alterations, which suggest impaired mitochondrial function, are confirmed by the electron microscopic observations of swollen neuronal mitochondria and an increased permeability of the organelles’ outer membranes (53). These factors all contribute to astrocytic swelling, as does an energy shortage–induced failure of the sodium pump (54). In addition, there is a local inflammatory response with complement and microglial activation and an increased release into cerebrospinal fluid (CSF) of a variety of cytokines (interleukin-1, interleukin-6, and interleukin-10) (56). These substances also contribute to the evolution of diffuse cerebral edema. The role of a trauma-induced upregulation of aquaporins, a family of transmembrane proteins that selectively allow the passage of water through the plasma membrane, in the formation of brain edema has not been fully clarified (56a).
The effects of traumatic brain injury on cerebral blood flow are critical to the development of cerebral edema. Early after severe traumatic brain injury, marked hypoperfusion develops with reduction in oxygen delivery and cerebral ischemia (57). Some 24 hours later cerebral blood flow increases, and there is uncoupling of cerebral blood flow and oxidative cerebral metabolism (1). Post-traumatic hyperemia is more common in infants and children than in adults and is probably the consequence of a loss of cerebral autoregulation (45). Hyperemia in turn contributes to massive brain swelling, which is not uncommon in infants and young children and has been termed malignant brain edema (58).
Anatomically, cerebral edema involves principally the subcortical white matter and the centrum semiovale. Less often, cerebral edema surrounds an area of contusion or an intracerebral hematoma. Brain swelling also can occur in response to the evacuation of a large extracerebral clot or after other major intracranial surgery (59).
When the additive effects of injury and brain swelling are severe, a self-perpetuating sequence develops, which can lead to further increases of intracranial pressure, with collapse of cerebral venules. This collapse in turn reduces cerebral perfusion and causes tissue hypoxia. This leads to further cerebral edema (60). A loss of selective permeability of cell membranes results, with increased loss of fluid from the vascular compartment into the parenchyma, thereby increasing cerebral swelling. Recovery has not been seen when intracranial pressure equals or exceeds mean systemic arterial pressure, at which point cerebral perfusion ceases.
Numerous changes occur secondary to cerebral edema. Herniation of the uncus over the tentorial edge compresses the midbrain and often occludes the posterior cerebral arteries. Edema and infarction of the occipital poles can occur, contributing further to supratentorial pressure and thus to herniation. Petechial hemorrhages develop in the midbrain and pons, with infarctions in the areas of the basal ganglia supplied by the anterior choroidal artery. A decrease in blood pressure, commonly seen with a severe injury, potentiates the vicious circle.
Clinical Conditions
Closed Head Injury
More than 90% of major pediatric head injuries are nonpenetrating and closed (i.e., no scalp wound exists). The clinical picture is highlighted by alterations in consciousness (14). As is the case for head injuries at all ages, boys are involved three times more often than girls.
Clinical Manifestations
When the injury is mild, initial unconsciousness is brief and is followed by confusion, somnolence, and listlessness. Vomiting, pallor, and irritability are common, and particularly in infants can occur in the apparent absence of an initial loss of consciousness. By definition, except for transient nystagmus or extensor plantar responses, neurologic signs are not observed in concussion. As a rule, a computed tomography (CT) scan clarifies the differential diagnosis of contusion, cerebral laceration, or other complications of closed head injury, and a lumbar puncture is not warranted without specific indications.
From 7% to 40% of children with mild head injuries have associated linear fractures of the skull (61). These fractures are most common in the parietal region. According to most authorities, such fractures do not, by themselves, affect the clinical course or prolong the period of morbidity; children with and without simple fractures have the same incidence of serious sequelae (14,61,62).
Electroencephalography (EEG), when performed soon after injury, can reveal striking abnormalities, such as generalized and focal slowing, prolonged reaction to hyperventilation, and even hypsarrhythmia. In milder head injuries, these changes tend to be transient. Taking into account the time elapsed between injury and recording, Mizrahi and Kellaway found that the degree of EEG abnormality correlated with the severity of the injury (63). Nevertheless, the EEG does not predict the development of post-traumatic epilepsy, and there is no correlation between the appearance of the EEG immediately after the head injury and development of early post-traumatic epilepsy (64). Proton resonance spectroscopy appears to be more valuable in predicting outcome. In the experience of Ashwal and coworkers, the presence of lactate had a poor prognosis in that 91% of head-injured infants and 80% of head-injured children with poor outcomes had a lactate peak; conversely, none of the infants and children with good outcome showed the presence of lactate (65).
In major closed head injuries, consciousness is interrupted more profoundly and for longer periods than in minor head injuries, and focal neurologic signs point to localized brain contusion. The clinical picture in such cases is outlined in Table 9.5 (66). Generally, the greatest neurologic deficit is found at the time of injury. New neurologic
signs appearing subsequently indicate progressive brain swelling or, if localized, indicate secondary intracranial hemorrhage, vasospasm, or thrombosis. The duration of coma depends on the site and severity of injury.
signs appearing subsequently indicate progressive brain swelling or, if localized, indicate secondary intracranial hemorrhage, vasospasm, or thrombosis. The duration of coma depends on the site and severity of injury.
TABLE 9.5 Clinical Findings in 4,465 Children with Major Closed Head Injuries | ||||||||||||||||||||||||||||||||||||||||
---|---|---|---|---|---|---|---|---|---|---|---|---|---|---|---|---|---|---|---|---|---|---|---|---|---|---|---|---|---|---|---|---|---|---|---|---|---|---|---|---|
|
The clinical picture follows one of several courses (67). Many children die without recovering consciousness. In a smaller group of patients, coma persists. Prognosis for survival is relatively good in children alive 48 hours after injury. In one-half of the surviving children, consciousness is regained in less than 24 hours. Recovery is often complete or nearly complete, although transient sequelae are not unusual. These include CSF leakage often complicated by secondary meningitis, post-traumatic epilepsy, and the development of a carotid artery–cavernous sinus fistula. Communicating hydrocephalus resulting from subarachnoid bleeding more commonly follows perinatal trauma and is discussed in Chapters 5 and 6.
Nonaccidental trauma
Child abuse is the most common cause of head injury in infants younger than 2 years of age. In the experience of Duhaime and coworkers, in 24% of infants admitted to hospital with head injuries the injuries were presumed to have been inflicted, and in another 32% the injuries were suspicious for child abuse (68). Although the term “shaken baby” has been used in the past, pathologic data collected on a larger Scottish series has made it clear that a whiplash shaking injury could be documented in only 6% of cases (69). The hyperextension and hyperflexion whiplashing forces injure the brainstem or upper cervical spinal cord with consequent acute respiratory failure, hypoxia, and brain swelling. Subdural bleeding was generally trivial. These pathologic findings are commensurate with the clinical presentation of collapse or respiratory arrest (70). Shannon and Becker also stressed the frequency of brainstem and spinal cord injuries in infantile child abuse (71).
The more commonly encountered clinical picture is characterized by depressed consciousness, increased intracranial pressure, seizures, and subdural and retinal hemorrhages. These symptoms are frequently accompanied by other nonaccidental injuries and an inconsistent or unreliable medical history. This constellation of symptoms has been termed the shaken impact syndrome. The encephalopathy developed acutely in 53% of the Scottish cases, subacutely in 19%, and chronically in 22% (69). The less acute the presentation, the better is the outcome with respect to long-term morbidity. In the series of Bechtel and coworkers, the clinical picture of nonaccidental head injury could be distinguished from that seen in accidental head injury by more frequent bilateral retinal hemorrhages, a greater likelihood of presenting with an altered mental state and seizures, and a lesser incidence of scalp hematomas (71a).
Pathologic examination of the brain of infants subjected to nonaccidental head injury disclosed skull fractures in 36%, acute subdural bleeding in 72%, and retinal hemorrhages in 71% (70). Severe hypoxic brain damage was present in 77%, and the usual cause of death, seen in 82%, was increased intracranial pressure. Eighty-five percent of the children had signs of impact to the head at autopsy, and 51% had significant extracranial injuries (70). The clinical presentation of infants who showed no signs of impact was of collapse or respiratory arrest; the pathologic picture did not differ from the majority of infants who showed evidence of impact (72).
There is considerable uncertainty as to the mechanism of brain damage in shaken impact syndrome, and according to Donohoe there is inadequate scientific evidence to come to a firm conclusion on most aspects of causation (73). Geddes and Plunkett (74) shared this skepticism and stressed that although most infants do indeed show signs of inflicted violence, serious injury or death from a low-level fall is possible, and contrary to Harding and coworkers, who believed that retinal hemorrhages result from rotational acceleration and deceleration forces (75), they doubted that shaking can induce the characteristic retinal hemorrhages.
Nonsurgical Treatment
Major Head Injury
A recommended sequence consists of the following four steps: a rapid initial evaluation, resuscitation, a more extensive secondary evaluation including radiologic assessment, and definitive treatment (1,45,76a). In many instances of major closed head injury, diagnosis of the injury is performed in parallel with emergency treatment.
Because in children the clinical condition can change rapidly and frequently, a high degree of alertness and preparedness is required of both medical and nursing attendants.
Neurologic Examination
Careful, but not elaborate, neurologic examination must be made and recorded, with particular emphasis on the state of consciousness; pupillary size, equality, and response to light; the extent and symmetry of spontaneous movements; and the reflex responses. Recording of blood pressure, pulse, and respiration is likewise essential. Caloric and optokinetic responses are useful for evaluating brainstem function. Some or all of these observations, especially the level of consciousness and motor activity, are principally of value when made serially at intervals that
can be as often as every 5 minutes. Such examinations allow trends away from or toward normal functioning to be detected, thus providing warning of any need to intervene surgically.
can be as often as every 5 minutes. Such examinations allow trends away from or toward normal functioning to be detected, thus providing warning of any need to intervene surgically.
TABLE 9.6 Pediatric Coma Scale | ||||||||||||||||||||||||||||||||||||||||||||||||
---|---|---|---|---|---|---|---|---|---|---|---|---|---|---|---|---|---|---|---|---|---|---|---|---|---|---|---|---|---|---|---|---|---|---|---|---|---|---|---|---|---|---|---|---|---|---|---|---|
|
Several pediatric modifications of the Glasgow Coma Scale (77), which is widely used for the assessment of head-injured adults, have been proposed. One example is presented in Table 9.6 (78).
The Glasgow Coma Scale measures three neurologic responses: eye opening, verbal response, and limb movement. Each response is given a score; the higher the score, the better is the condition of the patient.
Additionally, the extent of retrograde and post-traumatic amnesia should be recorded when possible. Spontaneous or provoked episodes of decerebrate activity or hypotonia are associated with a poor outcome (79); the length of pre- and post-traumatic amnesia is a useful indicator of the severity of the head injury (80).
Maintenance of Airway and Circulation
After the immediate evaluation of the child’s general condition, an adequate airway should be established and maintained. Airway obstruction is the most frequent cause of respiratory failure. Maintenance of patency requires suction of mouth or pharyngeal contents or endotracheal intubation followed, when necessary, by artificial respiration. Tracheostomy may be indicated to bypass mechanical obstruction of the airway caused by facial or mandibular injuries. Because an injury to the cervical spine must be presumed until proven absent, the neck must be stabilized while an airway is established. Arterial pressure is monitored by cannulation of a peripheral artery and maintained by administration of crystalloid, colloid, or blood products. Gas exchange also must be monitored.
Shock in closed head injuries is usually caused by blood loss elsewhere in the body. Rarely, it indicates damage to the medullary cardiovascular centers. Infants and young children have a higher incidence of shock than those in older age groups (1). In infants, in particular, subgaleal, subperiosteal, subdural, or extradural hemorrhages can be sufficiently extensive to induce shock. The injured brain is highly susceptible to episodes of systemic hypotension, in part because cerebral edema in conjunction with systemic hypotension lowers cerebral blood flow, and perhaps because an impairment of autoregulation of cerebral blood flow also occurs. Whatever the physiologic mechanism, when head injury is accompanied by systemic hypotension, the outcome is significantly worse (81).
After the initial emergency measures have been completed, the child’s neurologic status should be reevaluated. The importance of repeated observations of vital signs and neurologic status cannot be overemphasized. Slowing of the pulse rate and widening of pulse pressure often accompany an increase of intracranial pressure (Cushing effect) (see Chapter 11). An irregular respiratory pattern is also common after severe head injuries (82).
Neuroimaging Studies
CT of the head has revolutionized the clinical assessment of head injuries, and it is the most appropriate study for the assessment of intracranial complications, taking precedence over skull radiography and MRI. The need for and timing of such imaging studies depend mainly on the clinical findings. A suggested strategy is outlined in Table 9.7 (83). Some centers suggest that in children who show signs of brainstem compression and a Glasgow coma score of 3, precious time can be saved by foregoing CT and proceeding directly with exploratory surgery to remove a hematoma. Although emergency trephination may benefit the occasional patient, scanning is still the most important diagnostic study for the majority of children (84).
CT can delineate the presence and the extent of fractures and can demonstrate the presence of diffuse cerebral swelling and hyperemia of the brain (85), hemorrhages in the epidural and subdural space, and intracerebral hemorrhages (Fig. 9.4) (86).
In some injuries, the condition of the sinuses should be noted for evidence of a fracture, which makes the injury compound, or the presence of blood. Ethmoid and maxillary sinuses are pneumatized at birth, the sphenoid sinuses become pneumatized at approximately 5 years of age, and the frontal sinuses, the last to develop, are not visualized until 4 to 6 years of age (87).
TABLE 9.7 Management Strategy for Radiographic Imaging in Pediatric Patients with Head Trauma | ||||||||||||
---|---|---|---|---|---|---|---|---|---|---|---|---|
|
MRI usually contributes little to the initial management of major closed head injury. It is, however, more sensitive than CT in detecting an extraaxial hematoma, particularly a chronic subdural hematoma (88). Additionally, MRI provides better visualization of subacute and chronic contusions and of shearing lesions of the white matter such as result from child abuse (89). MR angiography (MRA), performed at a subsequent time, can be of use in delineating such vascular abnormalities as arteriovenous fistulae, venous sinus occlusion, and arterial occlusions.
One must guard against excessive complacency on the basis of a single normal CT obtained in the early hours after an injury because hemorrhagic complications can evolve subacutely after an interval of several hours or days. A repeat CT is indicated under the following circumstances: (a) when doubt exists about the presence of a mass lesion, (b) when intracranial pressure monitoring demonstrates an increase in pressure, (c) when a patient is unconscious despite an initial benign-appearing CT, and (d) when a contusion is accompanied by a neurologic deficit. A normal CT result also does not exclude the presence of cerebral edema. In the series of O’Sullivan and coworkers, 88% of head-injured patients with a Glasgow coma score of 8 or less and no evidence of cerebral edema by CT had increased intracranial pressure when recorded directly (90).
Fluid and Electrolytes
The appropriate fluid management of the hypovolemic head-injured patient remains controversial, although both clinical and experimental studies indicate that the most important goals are to correct any hypovolemia and prevent reduction of osmolality. A period of hyponatremia with natriuresis is a common response to brain injury (91). Hyponatremia can result from either a cerebral salt-wasting syndrome characterized by hypovolemia or from an inappropriate excess of antidiuretic hormone that causes water retention (syndrome of inappropriate
antidiuretic hormone secretion [SIADH]). Whereas the cerebral salt-wasting syndrome is treated by fluid and salt supplementation, SIADH requires fluid restriction. In the majority of patients, hyponatremia during the first week after a head injury is caused by excessive antidiuretic hormone, whereas hyponatremia that develops later probably results from chronic salt-wasting (92). Berkenbosch and colleagues advocated the measurement of urine output to distinguish between the two conditions. In SIADH, urine output is reduced, and at times there is a frank oliguria, whereas cerebral salt-wasting syndrome is marked by polyuria (93).
antidiuretic hormone secretion [SIADH]). Whereas the cerebral salt-wasting syndrome is treated by fluid and salt supplementation, SIADH requires fluid restriction. In the majority of patients, hyponatremia during the first week after a head injury is caused by excessive antidiuretic hormone, whereas hyponatremia that develops later probably results from chronic salt-wasting (92). Berkenbosch and colleagues advocated the measurement of urine output to distinguish between the two conditions. In SIADH, urine output is reduced, and at times there is a frank oliguria, whereas cerebral salt-wasting syndrome is marked by polyuria (93).
The relationship between fluid load, sodium balance, and intracranial pressure is unclear. The traditional view is that during the early post-traumatic period, there is a danger of overloading the patient with fluids, which can increase intracranial pressure and thus diminish the level of consciousness. Therefore, fluid intake for the first 2 to 4 days should be between 35% and 50% of the average normal daily fluid requirements, as long as urine volume remains adequate (94). This view has been challenged, and more recent work indicates that rapid, continuous infusion of hypertonic (3%) saline with a view of maintaining intracranial pressure below 20 mm Hg is safely tolerated and possibly improves the outcome of severely brain injured children without incurring a significant risk for renal failure or extrapontine myelinolysis (95). Most neurosurgeons currently recommend that hypertonic crystalloid solutions be given intravenously to maintain a normal intravascular volume and adequate cerebral perfusion pressure (96). Both clinical and experimental studies suggest that glucose-containing solutions should be avoided because these tend to increase cerebral lactic acidosis (97).
Despite the evidence of sodium retention in the early post-traumatic period, salt should be administered to avoid hypotonicity of extracellular fluids. A total of 30 mEq of sodium chloride per liter of calculated fluid requirements meets the usual electrolyte requirements. As a result of a catecholamine-induced intracellular potassium shift, hypokalemia can accompany severe head injuries (98). Although hypokalemia was documented in 7% of traumatic brain injuries admitted to the Boston Children’s Hospital, it corrected spontaneously, and in almost all instances did not require potassium supplementation (99).
Less commonly, hypernatremia and dehydration follow closed head injury. These are occasionally seen in a subfrontal injury that has caused hypothalamic damage and diabetes insipidus, or they are the result of a failure in the thirst response or inadequate hydration of an unconscious patient.
Coagulation Defects
A significant proportion of patients with head injuries severe enough to result in destruction of brain tissue show clinical and laboratory evidence of impaired coagulation (100). This results from structural damage, hypoxia, or elevated catecholamine and steroid levels. These patients have low fibrinogen levels, diminished amounts of factors V and VIII, and thrombocytopenia. Treatment can require emergency replacement of hemostatic factors with fresh-frozen plasma or recombinant activated factor VII (101). Disseminated intravascular coagulation was seen in approximately one-third of children evaluated within 2 hours of major head injury (102).
Seizures
Seizures can occur shortly after the injury or can appear after an interval of days to years. Seizures appearing during the acute stage can increase intracranial pressure by Valsalva effects. They can increase cerebral blood flow, cause the release of neuroexcitatory transmitters, and aggravate any preexisting hypoxia. They generally are managed with intravenous phenytoin (5 mg/kg loading dose). Intramuscular phenytoin, which can crystallize within muscles, is unreliable. Diazepam (0.1 to 0.3 mg/kg) and lorazepam (0.05 to 0.25 mg/kg) are suitable alternatives. Some authorities are loath to administer phenytoin to infants and toddlers, preferring carbamazepine. In adults, phenytoin appears to reduce the likelihood of early post-traumatic seizures but does not decrease the risk of late post-traumatic epilepsy (103,104). EEG monitoring is desirable to detect electrical seizures even when paralysis of the patient prevents overt seizures. The role of anticonvulsants in the treatment of post-traumatic seizures is discussed in Chapter 14. In view of the relative rarity of post-traumatic seizures, we do not advocate preventive anticonvulsant therapy, except in the presence of a major brain laceration. Chadwick is of the same opinion (103). For this purpose we prefer carbamazepine or valproate to phenytoin.
Increased Intracranial Pressure
Children tend to have a lower incidence of surgically treatable mass lesions than adults (less than 10% in a CT-verified series) but a higher incidence of increased intracranial pressure (105). Therefore, in the infant or child who has sustained a severe head injury, control of increased intracranial pressure becomes the most important problem of medical management. For this purpose, continuous pressure monitoring is necessary for the more seriously injured patients and for infants who are more likely to experience increased intracranial pressure (1,45,94). A variety of monitoring techniques are in use, each with its advantages and disadvantages. These include an indwelling ventriculostomy with direct monitoring from the ventricular system; monitoring from the subarachnoid, subdural, and epidural spaces (106); and fiber-optic monitoring by placement of a device into the frontal parenchyma (107). Of these methods, subdural monitoring using the Camino or Codman fiber-optic pressure
transducer is preferred at the University of California, Los Angeles, and the University of Washington. This allows an indirect, continuous measurement of intracranial pressure, which, when combined with the value of mean arterial blood pressure, permits calculation of cerebral perfusion pressure (cerebral perfusion pressure equals mean systemic arterial blood pressure minus intracranial pressure). When possible, estimation or measurement of cerebral blood flow allows detection of global cerebral ischemia, which generally worsens the clinical course and outcome (108). Focal cerebral ischemia is more difficult to assess. After major head injuries, some regions of the brain may require higher levels of cerebral perfusion pressure to maintain adequate cerebral blood flow.
transducer is preferred at the University of California, Los Angeles, and the University of Washington. This allows an indirect, continuous measurement of intracranial pressure, which, when combined with the value of mean arterial blood pressure, permits calculation of cerebral perfusion pressure (cerebral perfusion pressure equals mean systemic arterial blood pressure minus intracranial pressure). When possible, estimation or measurement of cerebral blood flow allows detection of global cerebral ischemia, which generally worsens the clinical course and outcome (108). Focal cerebral ischemia is more difficult to assess. After major head injuries, some regions of the brain may require higher levels of cerebral perfusion pressure to maintain adequate cerebral blood flow.
In some head-injured children CSF drainage via a ventriculostomy is a useful adjunct for controlling elevated intracranial pressure in those patients whose ventricles can safely be cannulated. Ventriculostomy remains the most effective way to monitor intracranial pressure and treat elevated pressure via continuous or intermittent CSF drainage. In selected patients who do not have mass lesions but have elevated intracranial pressures that are refractory to all other therapies, treatment with controlled lumbar CSF drainage has been encouraging (109).
Continuous monitoring of jugular venous oxygen saturation using an indwelling fiber-optic catheter also has been suggested, but the usefulness of this technique in the pediatric population has yet to be assessed (1). Venous oxygen saturation in the adult should lie between 55% and 85%, and values below 45% indicate global cerebral ischemia (110).
Intracranial pressure is usually maintained below 15 mm Hg, although temporary increases to 20 mm Hg are often unavoidable and can occur in the course of nursing procedures. The initial step in lowering intracranial pressure is to induce hypocarbia by hyperventilation and reduce the carbon dioxide partial pressure (pCO2) to between 25 and 30 mm Hg. With profound hyperventilation and pCO2 values below 25 mm Hg, cerebral ischemia can develop (111).
Historically, hyperventilation has been part of the treatment algorithm for trauma patients with elevated intracranial pressures. This maneuver causes cerebral vasoconstriction, thereby decreasing cerebral blood flow and volume. The response to hyperventilation is rapid but short lived, and hyperventilation must be continued or increased to remain effective. Studies show that the prolonged and routine use of hyperventilation may be deleterious in some patients by exacerbating ischemic brain injuries. Therefore, in the absence of elevated intracranial pressure, we no longer recommend the routine use of hyperventilation. Substantial beneficial effects can be realized when refractory elevated intracranial pressure is treated by moderate hyperventilation, accomplished by maintaining the arterial carbon dioxide pressure between 28 and 35 mm Hg. This therapy can be administered safely, with careful monitoring to avoid the complications associated with prolonged, profound hyperventilation, notably cerebral ischemia (112,113). Should hyperventilation be ineffective in reducing increased intracranial pressure, other measures must be undertaken.
Raising the head between 0 and 30 degrees in the neutral, midline position is commonly performed after severe head injury. This maneuver facilitates cerebral venous drainage and thus theoretically decreased intracranial pressure. Feldman and coworkers showed in their randomized study of head position of the trauma patient that by elevating the head from 0 to 30 degrees, the intracranial pressure was decreased without a decrease in cerebral perfusion pressure of cerebral blood flow (114). When the head is raised approximately 60 degrees, there appears to be a deleterious effect on cerebral blood flow. However, these studies have not been confirmed for children. Thus, the most advantageous head position in a child with an intracranial pressure monitor (i.e., the best way to maximize cerebral perfusion pressure) can be determined by raising the head and observing the concomitant change in intracranial pressure and cerebral perfusion pressure.
Diuretics are used in a number of centers. Mannitol and urea are the most commonly used osmotic diuretics. Mannitol, given in amounts of 1.5 to 2.0 g/kg body weight in the form of a 20% solution, has a slower effect than urea, but maintains the lowered intracranial pressure at its minimum level for 2 to 4 hours. It has the disadvantage of leaking into the brain through areas where injury has damaged the blood–brain barrier and thus inducing local edema. In addition, by slowly diffusing into the intercellular space, mannitol carries water with it, thereby inducing a rebound effect. For these reasons diuretics are used mainly as a temporary measure to gain time while the patient and the operating room are being readied for surgery, while diagnostic studies are being performed, or for acute control of elevated intracranial pressure. Mannitol, furosemide, or both are favored in most institutions; however, there are few well-controlled studies to show their effectiveness in the pediatric population (45). One appropriate dosing regimen for furosemide is 0.5 to 1.0 mg/kg administered every 4 to 6 hours.
Several well-controlled clinical studies have failed, however, to demonstrate any significant effectiveness of corticosteroids in counteracting brain swelling in head-injured children. The agents did not improve outcome or lower intracranial pressure and in some cases may have been deleterious. For these reasons, corticosteroids are not routinely employed in the treatment of pediatric head injuries. A multicenter, randomized, placebo-controlled trial of corticosteroids after significant head injury in adults with a Glasgow Coma Score of 14 or less within 8 hours of injury actually demonstrated an increase in mortality with methylprednisolone (114a,114b).
The clinical effectiveness of various oxygen-derived free radical scavengers, when given to patients with severe head injuries within 8 to 12 hours of the injury, has not been established in terms of improving neurologic outcome (115). Agents that inhibit lipid peroxidation, block excitotoxicity, and block apoptosis or promote brain cell regeneration have been employed in various experimental situations, but as yet have no clinical application.
High doses of barbiturates, generally pentobarbital, have been advocated whenever intracranial pressure does not respond to other forms of therapy. However, there is no convincing evidence that barbiturate coma improves outcome, and the initial enthusiasm for this form of treatment has waned because barbiturates can cause myocardial depression (1,116). For this reason barbiturates require cautious use in children, especially those with hemodynamic instability. Currently, barbiturates are used as a last resort to help control intractable elevations of intracranial pressure and to help place patients who are in status epilepticus into EEG burst suppression. Under these circumstances, bedside EEG monitoring is mandatory.
Sedation with pharmacologically induced paralysis is often used in the treatment of the severely head-injured child. This reduces the increase in intracranial pressure associated with agitation, suctioning, and ventilation. In our institutions, sedation and paralysis is titrated with intracranial pressure. Intravenous narcotics in small doses by continuous or bolus infusion, short-acting benzodiazepines, and nondepolarizing muscle relaxants are used effectively for this purpose. Titration of short-acting drugs permits intermittent examinations and rapid reversal, when necessary. In nonventilated, awake, but intermittently confused patients, medication is used sparingly because the neurologic examination is the most important parameter for the management of the head-injured patient. Small boluses of narcotics and short-acting benzodiazepines are used in some institutions to sedate the agitated pediatric patient without a mass lesion, but this issue remains controversial among neurosurgeons and critical care physicians.
Induced mild hypothermia (cooling to 32°C to 33°C) in conjunction with hyperventilation (pCO2 between 25 and 30 mm Hg) and barbiturates (4 to 6 mg/kg intravenous thiopental, followed by 6 to 8 mg/kg per hour of continuous thiopental) has reemerged as another measure to lower increased intracranial pressure and increase cerebral perfusion by lowering metabolic demands (45). Hypothermia attenuates the increases of excitatory amino acids, decreases endogenous antioxidant consumption, and has antiinflammatory effects. The effectiveness of this measure in the pediatric population has not been clearly demonstrated (45). Preliminary results suggest that this method can improve neurologic outcome in younger patients, particularly those who were already hypthermic on admission. From these studies it is not clear whether this represents a beneficial effect of early hypothermia or a detrimental effect of passively warming a previously hypothermic patient (117).
Neurogenic pulmonary edema is a rare and potentially fatal complication of head injury. Its pathogenesis is unknown, but focal brainstem lesions, particularly in the region of the nucleus solitarius, can increase pulmonary arterial pressure and capillary permeability. Symptoms appear within the first day after injury and are managed with diuretics and positive end-expiratory pressure (118).
More detailed treatment schedules for the child with a major closed head injury are given by Jennett and Teasdale (119) and Bayir and colleagues (45). All infants with head injuries and all older children with severe head injuries, as defined by a Glasgow coma score of 8 or less, or who have sustained a major skull fracture should be seen by a neurosurgeon.
Minor Head Injury
The American Academy of Pediatrics issued guides for the management of minor head treauma in children older than age 2 years (120) and younger than age 2 years (121). These are summarized in Table 9.8. Considerable judgment is
required to avoid unnecessary hospitalization and expensive diagnostic studies, at the same time keeping in mind the possibility of post-traumatic complications that require emergency surgery. In general, children who have experienced only a momentary loss of consciousness are better managed at home. Parents are instructed to note at regular intervals the child’s state of alertness and ability to move his or her extremities. The parents should be told to contact the physician if diminished consciousness or limb weakness occurs.
required to avoid unnecessary hospitalization and expensive diagnostic studies, at the same time keeping in mind the possibility of post-traumatic complications that require emergency surgery. In general, children who have experienced only a momentary loss of consciousness are better managed at home. Parents are instructed to note at regular intervals the child’s state of alertness and ability to move his or her extremities. The parents should be told to contact the physician if diminished consciousness or limb weakness occurs.
TABLE 9.8 Guidelines for Management of Children with Minor Head Injuries | ||
---|---|---|
|
The role of routine CT in the management of the child with minor head injuries continues to be controversial. As indicated in Tables 9.7 and 9.8, the infant younger than 2 years of age or the child who sustains loss of consciousness should undergo CT. The younger the child, the lower the threshold should be for imaging studies. In the small infant the incidence of significant intracranial injuries is greater, and there is a higher likelihood of asymptomatic intracranial injuries (121). The cost-effectiveness of CT as a screening for safe discharge as compared with hospitalization for observation has been affirmed in a number of centers both in the United States and Europe (122,123,124). These studies have not addressed the costs of follow-up visits for false-positive imaging studies or the costs of return visits and subsequent hospitalization for a youngster who is handled with observation only (120). The use of skull radiography as a screening device for children with minor head injuries has serious limitations and should no longer be used inasmuch as a CT with bone windows to show the presence of fractures is superior diagnostically (122). According to some authorities, the presence of a linear skull fracture should not influence the decision about whether to send the child home; others suggest that a child found to have a skull fracture is at increased risk for an extradural hematoma and therefore should be observed in the hospital (125). We believe that whenever the fracture line crosses the groove of the middle meningeal artery or crosses the path of the sagittal or other major venous sinus, the possibility of an extradural hemorrhage is increased, so that the child should be hospitalized for the initial 24 hours. If the fracture involves an air sinus, checking for the next 10 days for signs of intracranial infection is recommended even if the child is not hospitalized.
Prognosis
There has been continuing improvement in terms of mortality and ultimate neurologic status in the outcome of severely brain-injured infants and children as defined by a postresuscitation Glasgow coma score of 8 or less (127). The outlook for full intellectual function in children experiencing minor head injuries (i.e., head injuries without associated neurologic manifestations) is generally excellent (128). The prognosis for major head injuries, although less certain, is far better in children than in infants or adults. As a rule, prediction of outcome with respect to the severity of ultimate disability is accurate in only 70% by the end of the first week after the injury (129). In the experience of the Traumatic Coma Data Bank, children younger than age 4 years who have sustained a severe head injury have a cumulative mortality of 62% during the first year after their injury. This compares with a mortality during the same period of 22% in children aged 5 to 10 years and 48% in adults (130). In this series, only 1 in 5 infants had a favorable outcome. These figures, published in 1992, should be contrasted with a mortality of 10% in a cohort gathered in the years 1994 to 1996 (127). A low postresuscitation Glasgow coma score and the presence of cerebral swelling, particularly when it is accompanied by a shift of midline structures, are indices for a poor outcome. These indices have not changed in the last few decades. The poor outcome in infants in all series probably reflects the higher incidence of child abuse and multiple injuries during the first year of life. Keenan and colleagues, working in North Carolina, found that 53% of serious or fatal head injuries incurred by infants younger than 2 years of age were caused by child abuse (131).
In a series of children treated for major head injuries at the Johns Hopkins Hospital and reported in 1983, 29% were normal on follow-up and 53% had returned to school with mild behavioral or cognitive problems. In the latter group, however, 61% had shown evidence of significant language delay, minimal cerebral dysfunction, and learning problems before the accident (132). The outcome tended to be worse in children who sustained prolonged coma. When outcome is stratified according to age, all studies show that morbidity as well as mortality are greater when the injury is experienced in infancy or in early childhood.
Generally, children with only behavioral abnormalities during the early post-traumatic period later achieve normal functioning. No significant improvement in cognitive function can be expected after the first 6 to 12 months after injury. Improvement in speech and motor function, however, can continue for several years (133,134). Decerebrate rigidity during the early postinjury phase, although associated with an early mortality in 25% to 50% of patients, by itself does not preclude functional survival; approximately one-half of survivors have a fairly good quality of life (134). Excessive daytime sleepiness is fairly common after head trauma, especially in adolescents. In some of these, notably when there has been an associated whiplash injury, sleep apnea is responsible; in others, the condition resembles narcolepsy (see Chapter 15) (135). In the experience of Guilleminault and coworkers, daytime sleepiness
can persist for months to years, and its duration correlates with the severity of the initial head trauma (135). Methylphenidate or amphetamine appear to be the most effective medications. A significant postural and intention tremor affecting primarily the upper extremities has been observed in a substantial number of children recovering from a serious head injury. In the series of Johnson and Hall, the tremor appeared within 2 months of the injury in 49% of their patients and between 2 and 12 months of the injury in 40%. It subsided spontaneously in 54% (136). Hyperekplexia and myoclonic twitches have also been seen (137). The cause of this complication is not clear, but based on radiouptake studies, it probably reflects striatal dopaminergic denervation (138).
can persist for months to years, and its duration correlates with the severity of the initial head trauma (135). Methylphenidate or amphetamine appear to be the most effective medications. A significant postural and intention tremor affecting primarily the upper extremities has been observed in a substantial number of children recovering from a serious head injury. In the series of Johnson and Hall, the tremor appeared within 2 months of the injury in 49% of their patients and between 2 and 12 months of the injury in 40%. It subsided spontaneously in 54% (136). Hyperekplexia and myoclonic twitches have also been seen (137). The cause of this complication is not clear, but based on radiouptake studies, it probably reflects striatal dopaminergic denervation (138).
TABLE 9.9 Rehabilitation of Head Injuries at Each Level of Consciousness | |||||||||||||||||||||
---|---|---|---|---|---|---|---|---|---|---|---|---|---|---|---|---|---|---|---|---|---|
|
A substantial proportion of survivors from major head injuries experience subsequent emotional and psychiatric disorders. These are covered in another section of this chapter.
Skull Fractures
Linear Fractures
The immature and more flexible skull of the child can sustain a greater degree of deformation than that of an adult. Most skull fractures are linear and asymptomatic and, in the older child, are readily diagnosed by CT using bone windows. In infants, fractures tend to be irregular, so that on plain skull films they are sometimes confused with a suture or wormian bone (Fig. 9.5). In early childhood the fracture can be diastatic and usually involves the lambdoid suture. The separation of the bones indicates that the intracranial pressure increased at the moment of impact.
Often a subperiosteal or subgaleal hematoma, termed cephalhematoma, in the newborn infant accompanies a linear skull fracture. Palpation of the hematoma may falsely lead the examiner to think a depressed skull fracture exists. Imaging studies disclose the underlying linear fracture. A small number of these hematomas calcify (see Fig. 6.1). There rarely is an indication for aspiration of a traumatic hematoma, and insertion of a needle or drain only increases the risk of introducing an infection into the hematoma cavity (see Chapter 6 for further discussion of this complication).
Closed linear fractures generally heal in 3 to 4 months and, except for breaks crossing the path of major vessels or entering the paranasal sinuses, do not require special therapy or observations.
An infrequent complication of a closed head injury with a linear fracture in an infant is the diastatic or growing fracture (139). It occurs in less than 1% of all fractures and usually represents a complication of a serious head injury (140). It occurs when the fracture tears the dura causing the arachnoid to be trapped in the fracture (16). Rare after age 3 years, it is thought to be more common in victims of child abuse, but its appearance is not confined to victims of nonaccidental trauma (140). Dural tears responsible for a growing fracture occur mostly in the parietal area; their presence, unrecognized because the scalp is intact, leads to the development of a CSF-filled cyst between the cortex and the overlying bone. At the same time, the bone edges along the fracture do not unite, apparently prevented by their direct contact with fluid. The bone is resorbed, so that plain skull radiography or CT scans taken after an interval of several months show an irregular bone defect with scalloped edges that is an elliptical erosive cranial defect (139).
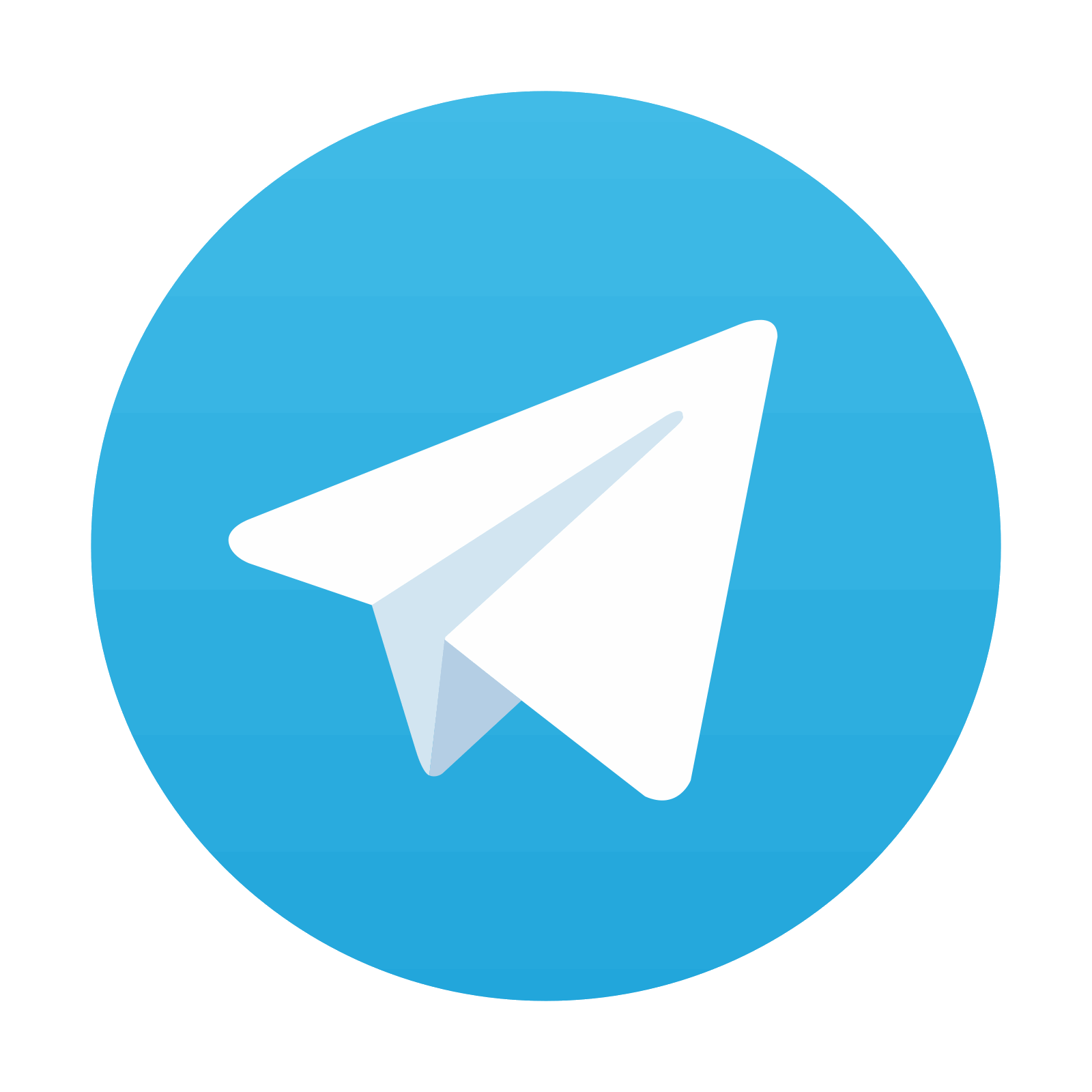
Stay updated, free articles. Join our Telegram channel
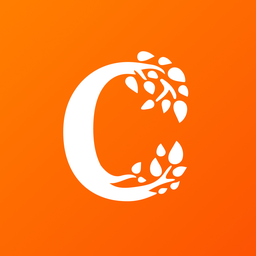
Full access? Get Clinical Tree
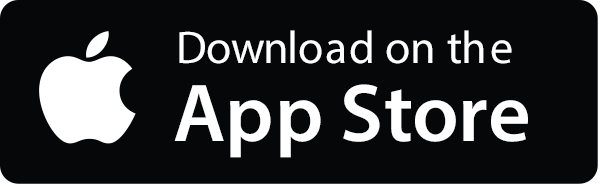
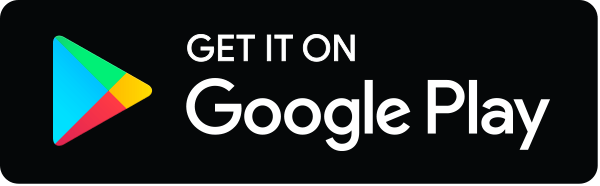
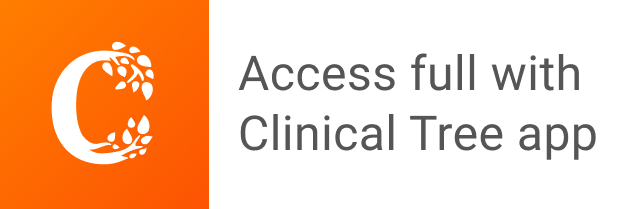