Chapter 9 Sensory Receptors and the Peripheral Nervous System
The ongoing activity and output of the central nervous system (CNS) are greatly influenced, and sometimes more or less determined, by incoming sensory information. An example is our constant awareness of the position of our limbs in space and the use of this awareness in guiding movements. The basis of this incoming sensory information is an array of sensory receptors, * cells that detect various stimuli and produce receptor potentials in response, often with astonishing effectiveness. Rod photoreceptors, for example, can produce measurable responses to single photons (see Chapter 17), and olfactory receptors may be able to respond to single odorant molecules (see Chapter 13); auditory and vestibular receptors can respond to almost unimaginably small mechanical deflections (see Chapter 14). The physiological processes employed by sensory receptors turn out to be gratifyingly similar to those found in synapses.
This chapter considers some general principles of the anatomy and physiology of sensory receptors. The emphasis is on the general receptors of the body—the principal purveyors of sensory information to the spinal cord (see Chapter 10 for what happens next). Specialized receptors, such as those of the eye, ear, mouth, and nose, are described in more detail in later chapters.
Receptors Encode the Nature, Location, Intensity, and Duration of Stimuli
Each Sensory Receptor Has an Adequate Stimulus, Allowing It to Encode the Nature of a Stimulus
Many Sensory Receptors Have a Receptive Field, Allowing Them to Encode the Location of a Stimulus
Specific wiring patterns in ascending sensory pathways and in the cerebral cortex preserve information about the nature of a stimulus. In some sensory systems, individual receptors convey information not only about the nature of a stimulus but also about its location. That is, in addition to adequate stimuli, individual receptors may have receptive fields, particular areas in the periphery where application of an adequate stimulus causes them to respond. The receptive field of a cutaneous receptor, for example, is an area of skin where its receptive endings reside (Fig. 9-1). The receptive field of a retinal photoreceptor is some small location in the outside world whose image falls on the particular spot on the retina where that photoreceptor is located. This preservation of spatial information is apparent in the CNS as the common occurrence of distorted maps (see Fig. 3-30). Even arrays of receptors with no obvious spatial domain to map may have systematic representations of some other parameter, such as the mapping of sound frequencies in the cochlea and in auditory cortex (see Fig. 14-19).
Receptor Potentials Encode the Intensity and Duration of Stimuli
The nature and location of a stimulus are indicated by the identities of the receptors that respond. To a great extent the intensity and duration of a stimulus are indicated by the size and duration of the receptor potentials produced—more intense stimuli produce larger receptor potentials, and longer stimuli cause longer receptor potentials (Fig. 9-2). There is a bit more to the intensity-duration story than this, however. Some sensory systems include more sensitive and less sensitive receptors (e.g., the rods and cones of the retina), so increasing intensity may be signaled in part by the identities of the activereceptors. In addition, as discussed in the next section, some sensory receptors produce only brief receptor potentials, even in response to maintained stimuli.
Most Sensory Receptors Adapt to Maintained Stimuli, Some More Rapidly Than Others
Nearly all receptors show some adaptation, which means they become less sensitive during the course of a maintained stimulus. * Those that adapt relatively little are called slowly adapting and are suitable receptors for such things as static position. Those that adapt a great deal typically do so quickly and are called rapidly adapting; they are better suited to indicate change and movement of stimuli (Fig. 9-3). Some rapidly adapting receptors (e.g., the Pacinian corpuscles shown in Fig. 9-8) do so completely and signal only the beginning and end of a stimulus; others continue to respond, but at a diminished level, throughout a stimulus. Adaptation is usually a property of one or more parts of the receptor’s membrane: Ca 2+ ions entering through transduction channels, for example, set in motion biochemical processes that decrease the sensitivity of some receptors. In addition, various accessory structures may modify the physical stimulus before it reaches the sensory ending, as in the case of the multilayered capsules of Pacinian corpuscles.
Adaptation is a receptor-level process, but the CNS also has ways to regulate the sensitivity of receptors on a moment-to-moment basis. One such mechanism is the control of other structures related to receptors—for example, controlling the amount of light reaching the retina by regulating pupil size, or controlling the muscle fibers in stretch receptors (see Fig. 9-15). Another mechanism used is efferent projections from the CNS that synapse on receptor cells themselves; these are prominent in the inner ear (see Chapter 14) but occur in some other sensory systems as well.
Sensory Receptors All Share Some Organizational Features
Although their morphologies vary widely, all receptors have three general parts: a receptive area, an area rich in mitochondria (near the receptive area), and a synaptic area, where the receptor’s message is passed toward or into the CNS (Fig. 9-4). The receptive area may have specializations suited to the adequate stimulus, as in the case of photoreceptors, which have an elaborately folded array of photopigment-bearing membrane; in other cases, there are no obvious specializations in this area. The area rich in mitochondria is either immediately adjacent to the receptive membrane or nearby and is presumed to supply the energy needs of the transduction process. In some receptors the synaptic area may be far removed from the other two, as in the case of a cutaneous mechanoreceptor with its receptive endings in the skin and its synaptic terminals in the spinal cord or brainstem.
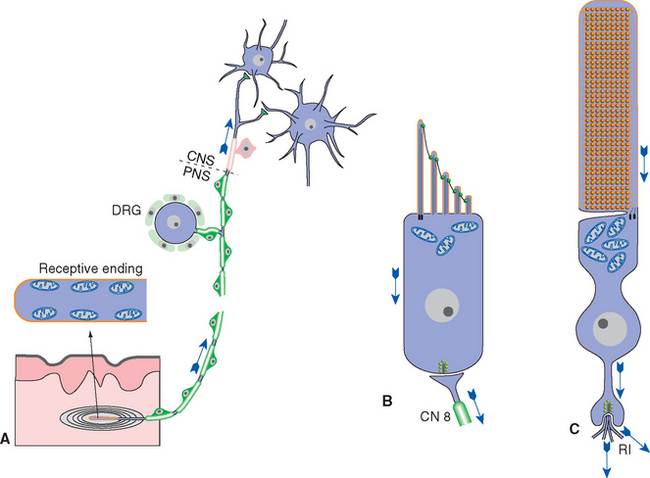
Figure 9-4 General organization of sensory receptors, as illustrated by a somatosensory receptor (Pacinian corpuscle, A), a hair cell of the inner ear (B), and a retinal rod photoreceptor (C). Each has a receptive area (orange) and mitochondria nearby. The receptive ending of the Pacinian corpuscle has no obvious anatomical specializations but is surrounded by a layered capsule. The microvillar projections of the hair cell contain mechanosensitive channels (see Chapter 14), and the receptive area of the rod contains a collection of pigment-studded membranous disks (see Chapter 17). Somatosensory receptors make synapses far away in the CNS, whereas hair cells and photoreceptors make synapses nearby on peripheral endings of vestibulocochlear nerve fibers (CN 8) or on retinal interneurons (RI), respectively. DRG, dorsal root ganglion.
Sensory Receptors Use Ionotropic and Metabotropic Mechanisms to Produce Receptor Potentials
Sensory receptors transduce (Latin for “lead across”) some physical stimulus into an electrical signal—a receptor potential—that the nervous system can understand. Receptor potentials, like other electrical signals across neuronal membranes, are produced by the opening or closing of ion channels. (The only known exception in primates is the taste receptors that detect saltiness, as discussed in Chapter 13.) In many ways, most sensory receptors can be thought of as analogous to postsynaptic membranes, and their adequate stimuli as analogous to neurotransmitters (Fig. 9-5). Just as postsynaptic membranes use ionotropic and metabotropic mechanisms to produce postsynaptic potentials, almost all known transduction mechanisms involve ion channels whose conductance is affected either directly by a stimulus (as in transmitter-gated ion channels) or indirectly by way of a G protein–coupled mechanism. As in the case of synapses, some sensory receptors (e.g., somatosensory mechanoreceptors) produce depolarizing receptor potentials when stimulated, and others (e.g., photoreceptors) produce hyperpolarizing receptor potentials. Auditory and vestibular receptors can produce either, depending on the details of the stimulus.
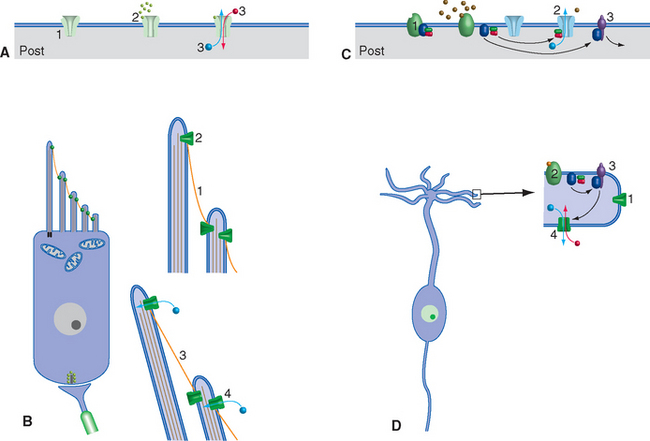
Figure 9-5 Similarities between the general mechanisms of postsynaptic potentials and receptor potentials; a vestibular receptor cell (see Chapter 14) and an olfactory receptor cell (see Chapter 13) are used as examples, but almost all receptors use mechanisms that are similar in principle. A, Rapid synaptic transmission involves ligand-gated (ionotropic) channels (1) that bind neurotransmitter (2) and then change permeability (3). B, Cochlear and vestibular hair cells have channels that are anchored to the cytoskeleton and to one another. When there is little tension (1) in the filamentous proteins interconnecting the channels, they spend much of their time closed (2). Increasing the tension (3) by deflecting the microvilli bearing the channels causes direct opening of the channels (4) and depolarizing current flow. C, Slow synaptic transmission involves G protein–coupled (metabotropic) receptors (1). Dissociation of the G protein in response to neurotransmitter binding may affect ion channels either directly (2) or indirectly via enzymatic cascades (3). D, Olfactory receptor cells contain normally closed channels (1) and G protein–coupled receptors for odorants (2). Dissociation of the G protein activates an enzyme (3, adenylate cyclase), which catalyzes the production of a second messenger (cyclic AMP) that in turn opens the transduction channels (4), causing a depolarizing receptor potential.
All Sensory Receptors Produce Receptor Potentials, but Some Do Not Produce Action Potentials
Receptor potentials, like postsynaptic potentials, are focally produced events that spread electrotonically. If a particular sensory receptor is physically small relative to its length constant—that is, if it contacts the next cell in its neuronal pathway close to the site of transduction—the receptor potential itself can adequately modulate the rate of transmitter release at the synaptic terminal. This in turn causes a postsynaptic potential, and typically a change in action potential frequency, in the second cell (Fig. 9-6A). This is the case for many receptors, prominently including photoreceptors and auditory and vestibular receptors, all of which produce receptor potentials but no action potentials. Some receptors, however, must convey information over long distances (e.g., from a big toe to the spinal cord), even though the receptor potential dies out within a few millimeters. In such cases, most of the receptor, beginning near the site of transduction, is capable of propagating action potentials. The action potential frequency is then modulated by the receptor potential (Fig. 9-6B). Receptor potentials that directly cause changes in action potential frequency are also called generator potentials. All somatosensory receptors of the body operate in this manner, as do olfactory receptors and many visceral receptors. In vertebrates, all produce depolarizing receptor potentials, and an increased frequency of action potentials, in response to stimulation.
Somatosensory Receptors Detect Mechanical, Chemical, or Thermal Changes
Somatosensory receptors include an assortment of mechanoreceptors, thermoreceptors, and nociceptors. All are pseudounipolar neurons with cell bodies in a dorsal root or cranial nerve ganglion, a central process that terminates in the spinal cord or brainstem, and a peripheral receptive ending in someplace like the skin, a muscle, or a joint (Fig. 9-1). A great deal is understood about the moment-to-moment responses of many of these receptors, mostly from animal studies, but also in large part because it is possible to record from the axons of single receptors of human volunteers, using a process called microneurography (see Fig. 9-14, insets).
Cutaneous Receptors Have Either Encapsulated or Nonencapsulated Endings
The skin and adjacent subcutaneous tissues are richly innervated by a wide variety of sensory endings. These endings can be divided broadly into encapsulated and nonencapsulated receptors (Table 9-1), depending on whether a capsule surrounds the ending.
A bewildering variety of encapsulated receptors have been described in the past, and an equally bewildering variety of mostly eponymous names have been attached to them. However, these classifications are just variations on two common themes: receptors with layered capsules and receptors with thin capsules. This chapter describes only the best known of these. The function of the capsule is not known for all encapsulated receptors, but in at least some instances it serves as a mechanical filter, modifying mechanical stimuli before they reach the sensory ending. For example, receptors with layered capsules are rapidly adapting, due in large part to these mechanical properties of the capsules. The capsules also have barrier properties (discussed later in this chapter) that may be important in regulating the composition of the fluid surrounding the sensory endings contained within them.
Capsules and Accessory Structures Influence the Response Properties of Cutaneous Mechanoreceptors
In addition to mechanoreceptive free nerve endings, there are five other prominent types of mechanoreceptor found in the skin and adjacent subcutaneous tissue. Two are nonencapsulated endings with accessory structures, and three are encapsulated.
Endings around hairs vary in their degree of complexity. Those around the base of a cat’s whiskers are very elaborate, but those around most ordinary human body hairs are longitudinal neural processes or spiral endings that wrap around the base of the hair (Fig. 9-7). Bending the hair is presumed to deform the sensory ending, distort mechanically sensitive channels, and lead to the production of a generator potential. Most hair receptors are rapidly adapting; they respond well to something brushing across the skin but not to steady pressure. *
The second type of nonencapsulated receptor is the Merkel ending, which is found in both hairy and glabrous skin (Fig. 9-7). The ending is a disk-shaped expansion of a terminal of a sensory fiber, which is applied to the base of a specialized cell called a Merkel cell. A single fiber branches to innervate several Merkel cells, which usually occur in clusters. Each Merkel cell is situated in the basal layer of the epidermis and contains dense-cored vesicles in what looks like a synaptic ending onto the sensory terminal. This apparent synapse naturally led to the hypothesis that the Merkel cell is sensitive to deformation and uses this synapse to pass information about mechanical stimuli along to the nerve ending (which would make it the only known example of a somatosensory receptor that is not part of a dorsal root ganglion cell). However, the available evidence is inconclusive, and the role of the Merkel cell is currently uncertain. Recordings from these sensory fibers have shown that Merkel endings are slowly adapting mechanoreceptors.
Meissner corpuscles are elongated, encapsulated endings in the dermal papillae of hairless skin just beneath the epidermis, oriented with their long axis perpendicular to the surface of the skin (Fig. 9-8). The encapsulation consists of a thin outer capsule and a layered stack of Schwann cells within the capsule, with the Schwann cells oriented perpendicular to the long axis of the capsule (like a stack of pancakes). One or more myelinated fibers approach the base of the corpuscle, lose their myelin, and wind back and forth between the stacked cells within the capsule. These are rapidly adapting receptors, and it is assumed that the capsule and the layered Schwann cells within it are important in determining the degree of adaptation. Vertical pressure on a dermal papilla compresses the nerve endings between the stacked capsular cells of a Meissner corpuscle, whereas pressure on a neighboring papilla is not nearly so effective. Meissner corpuscles are abundant in the skin of fingertips, and they and Merkel endings are largely responsible for our ability to perform fine tactile discriminations with our fingertips (Fig. 9-9; see also Fig. 9-18). Most animals have their own species-specific patterns of distribution of receptors, with specializations in functionally important parts of the body. Rats and cats have elaborate arrays of receptors surrounding their whiskers and large areas of somatosensory cortex devoted to their whiskers. Elephants mind their trunks. Other animals have other patterns (Box 9-1).
< div class='tao-gold-member'>
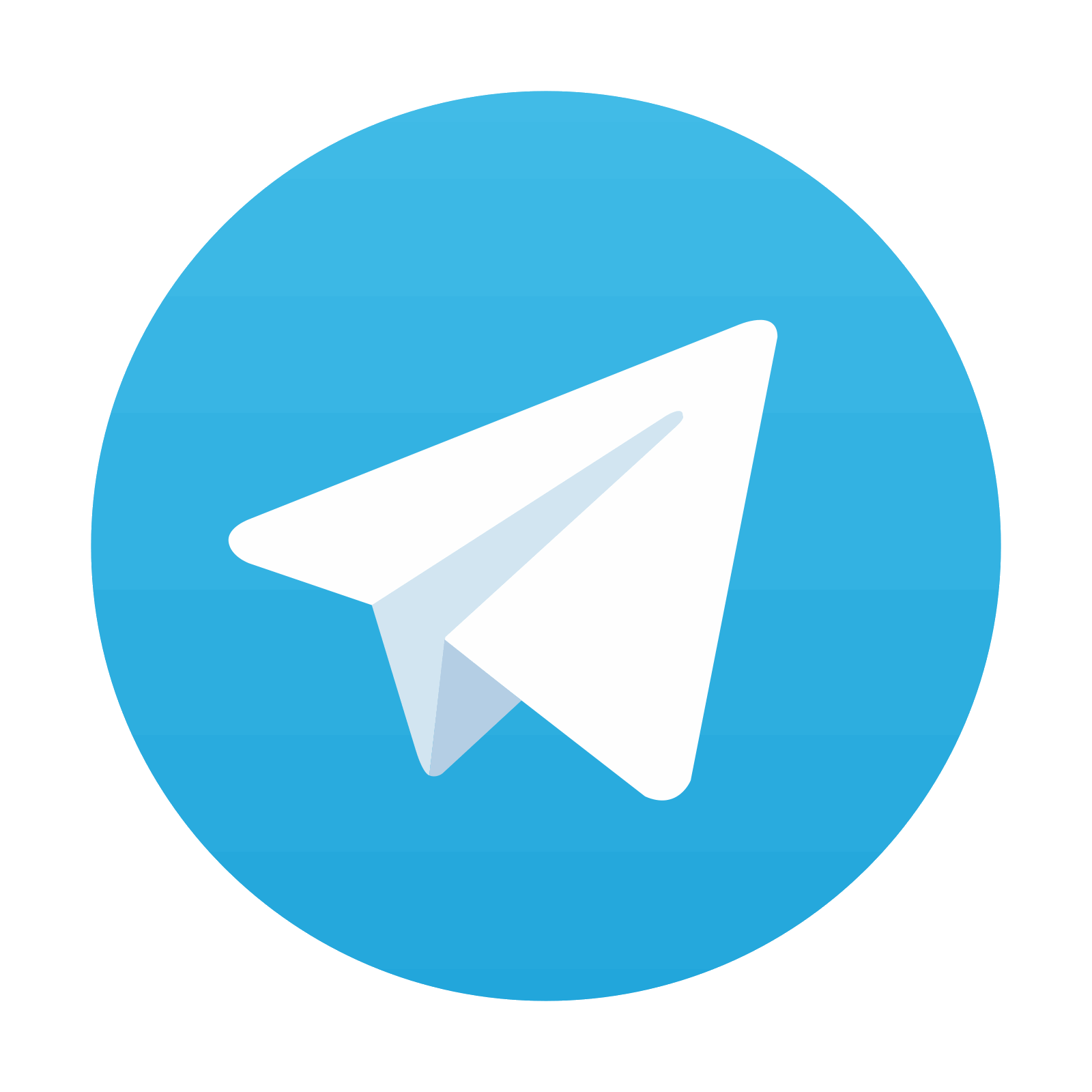
Stay updated, free articles. Join our Telegram channel
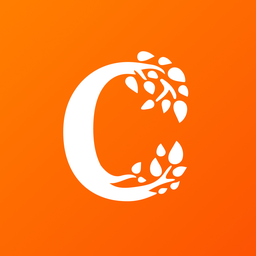
Full access? Get Clinical Tree
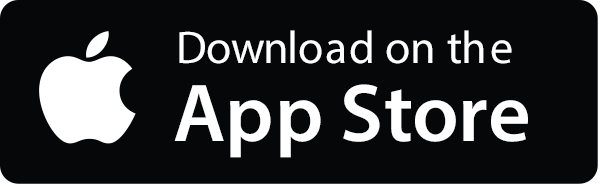
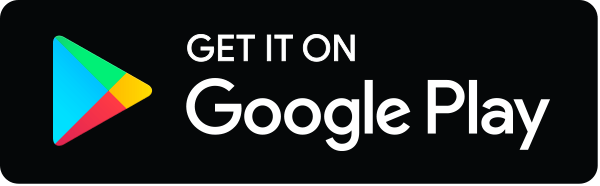