Both sleep and arousal are active processes mediated by specific brain regions and neurotransmitter systems.
Sleep can be divided into two phases, nonrapid eye movement (non-REM) and REM sleep, the latter being characterized by brain activity resembling that observed during the waking state.
The different physiologic functions of sleep are unknown; a role in memory consolidation is likely.
The initiation of non-REM sleep is controlled in part by GABAergic neurons in the preoptic/anterior hypothalamic area; the initiation of REM sleep is controlled by cholinergic cells in the pontine tegmentum.
Sleep is controlled both by circadian rhythms and by the homeostatic drive produced by periods of wakefulness. Adenosine is an important mediator of the homeostatic drive for sleep.
The suprachiasmatic nucleus is the primary pacemaker for the circadian regulation of sleep and other physiologic processes, in particular, for entraining circadian rhythms to environmental light.
Circadian rhythms are produced, in part, by the complex transcriptional regulation of “clock” genes that have been conserved throughout evolution.
Sleep disorders are a significant cause of morbidity. A major advance is the finding that decreased expression of the hypothalamic neuropeptide orexin (also called hypocretin) causes most human narcolepsy.
Benzodiazepines and similarly acting drugs, which are positive allosteric modulators of GABAA receptors, are the most common pharmacologic treatments for insomnia.
General anesthetics, comprising diverse classes of compounds, induce a non-REM sleep-like state characterized by amnesia, analgesia, immobility, and hypnosis. Most general anesthetics act, at least in part, by facilitating inhibitory ion channels (including ligand-gated channels) or inhibiting excitatory ion channels.
Defining sleep and arousal is not a simple matter. Sleep is clearly different from hibernation, coma, or states produced by general anesthesia. The first part of this chapter presents a broad overview of the neurobiology of sleep and arousal states. The second part covers some of the major sleep disorders, the mechanisms of which are slowly being elucidated. The third part discusses the pharmacologic treatment of these disorders.
As with other behavioral states such as fear and reward (Chapters 15 and 16), sleep states, such as rapid eye movement (REM) and nonrapid eye movement (NREM) sleep, depend on specific neural circuits. However, certain phenomena that occur during sleep may also employ some of the circuitry that contributes to behavioral states during waking hours. Night terrors, for example, involve a partial arousal out of deep NREM sleep; patients exhibit powerful sympathetic activation including tachycardia, mydriasis, and diaphoresis, despite the fact that they remain asleep. In contrast, patients with REM behavior disorder may have bouts of violent behavior during REM sleep that are not accompanied by elevations in heart rate or blood pressure. Hence, states of sleep and arousal are complex and heterogeneous and cannot be explained by a simple rheostat model.
Before the beginning of the 20th century, sleep was conceptualized as a passive process; sleep and arousal were believed to reflect a continuous gradient of levels of consciousness. It was believed that the level of arousal corresponded to the number of neurons that were actively engaged in processing information, in much the same way that the illumination from a light bulb varies depending on the amount of electric current it receives. During the second half of the 20th century, however, it was discovered that distinct cell groups in the brainstem and forebrain are more active during sleep, demonstrating that the existing model was too simplistic.
An early clue that sleep is not merely a passive state but an active process emerged with the finding that particular brain regions must be intact for sleep to occur. It was discovered, for instance, that lesions of the anterior hypothalamus create a state of chronic insomnia, which suggested that activity in this region is necessary for sleep. In contrast, lesions involving the posterior hypothalamus create a state of hypersomnolence.
The advent of the electroencephalogram (EEG) in the 1930s permitted noninvasive assessment of brain function in humans and animal models. The EEG remains the central tool in studies of sleep and wakefulness. Accordingly, sleep states have been defined by EEG patterns. EEG uses scalp electrodes to detect electrical signals produced mainly by surface areas of the brain. The EEG has excellent temporal resolution, but because it integrates information coming from a very large number of neurons, it has very limited spatial resolution.
In 1948 it was discovered that stimulation of the reticular formation of the brainstem elicits EEG arousal that is independent of sensory afferent pathways. Thus, arousal began to be viewed as an intrinsic property of the brain mediated by specific cell groups rather than a simple correlate of increased sensory input. This change in perspective roughly coincided with the discovery that alertness and arousal have a circadian rhythmicity that is independent of the number of hours an individual sleeps. Studies of sleep-deprived individuals indicated that alertness increases in the midmorning from a nadir that occurs late at night despite a long period of continuous wakefulness. Such findings demonstrated that arousal is not a simple function of previous rest.
In the late 1950s, cats with pontine lesions were reported to have REM sleep without the normal loss of muscle tone (atonia) that occurs in this sleep stage (and presumably keeps humans and most probably other animals from acting out their dreams). In these studies the lesioned cats walked and exhibited attack and defense behaviors while they were unresponsive to external stimuli and their EEGs were in a REM state. Subsequent histochemical studies of the brainstem suggested that REM is a complex state that is controlled by several neurotransmitter systems; these studies laid the groundwork for electrophysiologic experiments in the 1970s that showed that the onset of REM is generated by cholinergic cells in the pontine tegmentum (PT) and that inputs from the noradrenergic locus ceruleus (LC) and serotonergic raphe nuclei (RN) inhibit REM (Chapter 6). These findings in turn led to the development of an influential reciprocal interaction model of REM regulation, which is described later in this chapter.
Normal sleep exhibits fairly stereotypic patterns as measured by the EEG 13–1. In adults, in the first hour of sleep, brain activity descends through a series of NREM stages into deep sleep. NREM stage 1 occurs during the transition between wakefulness and sleep and is defined by the presence of background theta activity (4–7 Hz) that comprises more than 50% of each 30-second epoch. NREM stage 2 is composed of a background rhythm of theta activity with superimposed burst–pause waveforms such as K-complexes (high-amplitude, slow-frequency electronegative waves followed by electropositive waves) and spindles (brief bursts of 7–14 Hz activity). NREM stages 3 and 4, together referred to as slow-wave sleep, are defined by the presence of slow-wave (0.1–4 Hz), or delta, sleep activity that comprises 20% and 50% of each epoch of sleep, respectively. The predominance of low-frequency activity in slow-wave sleep indicates greater synchrony in neuronal firing and leads to a summed polarization and depolarization large enough to be recorded by the EEG.
After approximately 90 minutes of NREM sleep, the brain’s electrical activity reascends through the same stages toward the first cycle of REM sleep. REM sleep is characterized by low-amplitude, mixed-frequency and desynchronized EEG activity that appears similar to the EEG of the normal waking state 13–1. The desynchronization of the EEG results from individual neurons being in many different states of activity. This EEG pattern is accompanied by REMs—hence the name REM sleep—and profound relaxation of limb muscles. The NREM–REM sleep cycle repeats itself four or five times in the course of the night, with decreasing amounts of slow-wave sleep and increasing amounts of REM sleep in successive cycles 13–2.
Intermittent awakenings are part of normal sleep and may serve an adaptive function; for example, they may enable changes in posture to minimize circulatory pooling or nerve compression, or appraisals of the environment to guard against possible threats. Humans are unaware of most of these brief awakenings because they experience retrograde amnesia for several minutes after resuming sleep. A common misperception is that dreams occur only during REM. In fact, dreams occur during all stages of sleep but tend to be more dramatic, varied, and vivid in the latter part of the night during REM.
Newborns spend 20 hours per day in sleep. These hours are characterized by an incomplete segregation between REM-like (or active) sleep and NREM-like (or quiet) sleep. By 6 months of age, REM and NREM sleep can be reliably distinguished. During the first 3 years of life, humans begin to segregate sleep and waking until they become two distinct, consolidated periods. During adolescence a precipitous drop in slow-wave sleep activity occurs. With advancing age there is a gradual decline in total hours of sleep together with an increase in the number of awakenings. The sixth and seventh decades of life are characterized by a decline in total slow-wave sleep activity, which correlates with a decline in EEG amplitude. Elderly people experience more awakenings, complain more often of insomnia, and shift more of their sleep time to the day in the form of naps. Older people have a propensity to fall asleep at earlier hours and to have more early morning awakenings (phase advance of circadian rhythms). The decline in total sleep time in healthy older subjects is relatively modest, however, with more severe declines often related to medical conditions, pain, or depression.
Each sleep stage is accompanied by specific physiologic changes. Slow-wave sleep is associated with a reduced metabolic rate and a decline in brain and body temperature. Electrophysiologic features of all four NREM stages of sleep include increased firing of sleep-active centers in the anterior hypothalamus, hyperpolarization of thalamocortical neurons, and burst–pause activity driven by the reticular thalamic nucleus. Broad systemic features of stages 1 to 4 include behavioral quiescence, low muscle activity, reduced blood pressure, reduced heart and respiration rates, reduced cortisol and thyroid hormone levels, and increased growth hormone, testosterone, prolactin, insulin, and glucose levels (Chapter 10).
REM sleep, in contrast, is a unique state of arousal characterized by brain electrical activity, cerebral blood flow, and brain glucose metabolism similar to that of wakefulness. REM sleep is accompanied by a generalized muscular atonia except for the extraocular muscles (thus the REMs) and the diaphragm. Clitoral or penile tumescence, phasic bursts of eye movements, and alternating acceleration and deceleration of heart and respiratory rates also characterize REM.
Electrophysiologic recordings during REM sleep have documented tonic cerebral activity, synchronized firing of hippocampal neurons in the theta frequency range, and phasic pontogeniculooccipital (PGO) spikes originating in the pons. Several limbic structures and regions of the hypothalamus and brainstem reach their highest firing rate during REM. Positron emission tomography (PET) studies in humans have shown that limbic structures such as the amygdala become metabolically active during REM sleep. Interestingly, despite the pattern of cerebral activation, the threshold for arousal from REM sleep is higher than that from NREM sleep.
The need to sleep is universal among mammals, birds, and reptiles and has been conserved in evolution. It has even been reported in Drosophila. Despite the obvious disadvantages of sleep, such as reduced vigilance against predators, it is necessary to sustain life. It is claimed that total sleep deprivation in rodents can result in death (although this result has been contested). Another striking observation that supports the importance of sleep has come from marine mammals, which must remain awake constantly in order to surface every few minutes for air. These mammals sleep unihemispherically; that is, one cerebral hemisphere exhibits sleep patterns detectable by EEG, while the other hemisphere remains awake. The persistence of sleep states in animals that must remain awake to breathe is a powerful indication that sleep serves an essential function.
Despite intense scientific research, no theory has convincingly explained the function of sleep. Questions regarding the amount of sleep that is needed, the stage of sleep that is most restorative, and the interventions that best promote healthy sleep remain unanswered. The popular belief that sleep is needed to restore the integrity of body functions makes some intuitive sense. Yet, this theory is accompanied by a false assumption that sleep is an inactive state. Because quiet wakefulness and sleep differ very little in terms of energy expenditure, it is unlikely that sleep and its attendant reduction in vigilance were conserved in evolution simply to save energy. Furthermore, no compelling evidence suggests that tissue rebuilding or an increase in protein synthesis is associated with sleep. Many types of external and internal stimuli, including ambient temperature, darkness, food intake, exercise, and endogenous immune factors, affect sleep, but none of these variables has provided clues to the core function of sleep.
Many hypotheses have been used to explain the functions of REM sleep, but these frequently focus on a particular feature of REM, such as PGO spikes, and ignore other features. One prominent and intriguing hypothesis is that REM is needed to enhance learning or to discard irrelevant memory traces. While sleep may be important for enhancing the consolidation of memories (see below), the evidence in support of a specific role for REM in this process remains incomplete. Another interesting hypothesis, which arises from observed relationships between the proportion of sleep spent in REM by species based on their degree of maturity at birth, is that REM is needed for early neurodevelopment in species, such as our own, characterized by marked immaturity at birth. However, this hypothesis does not adequately explain the persistence of REM in adulthood and the increased amount of REM sleep that occurs after sleep deprivation. Current hypotheses also fail to explain the functional significance of alternations between REM and NREM sleep cycles, although it appears that the length of these cycles in mammals correlates closely with brain size. Consequently, the function of sleep may relate to crucial molecular and cellular processes not yet adequately understood.
There is increasing evidence that sleep plays an important role in memory. Sleep in general seems to be important for memory processing: long-term memory consolidation and reconsolidation seem to occur during sleep. (These aspects of memory are discussed in greater detail in Chapter 14.) For example, during early nocturnal sleep when slow-wave sleep is predominant, hippocampus-dependent declarative memories are replayed, stabilized, and likely enhanced. On the other hand, late sleep, when REM sleep predominates, has been associated with amygdala-dependent emotional memory. There is also evidence suggesting a role for sleep in motor learning, specifically in post-training consolidation: a period of sleep after learning a motor task has been shown to improve performance. In such experiments, one group of subjects practices a task in the morning and is tested late in the day, while another group practices the task in the evening, is permitted to sleep, and then is tested after an equivalent time lapse as the “day-wake” group.
Several neural systems mediate the switching between wakefulness and sleep and between the different stages of sleep 13–3. These systems include the ascending reticular activating system (ARAS), the ventrolateral preoptic (VLPO) area, and the orexin/hypocretin system (Chapters 6 and 7).
13–3
A schematic drawing showing key components of the ARAS. A major input to the relay and reticular nuclei of the thalamus (blue pathway) originates from cholinergic (ACh) cell groups in the upper pons, the pedunculopontine tegmental (PPT) and laterodorsal tegmental (LDT) nuclei, which together are considered the pontine tegmentum. These inputs facilitate thalamocortical transmission. A second pathway (red) activates the cerebral cortex to facilitate the processing of inputs from the thalamus. This arises from neurons in the monoaminergic cell groups, including the tuberomammillary nucleus (TMN) containing histamine (His), the ventral tegmental area (VTA) containing dopamine (DA), the dorsal and median raphe nuclei containing serotonin (5HT), and the locus ceruleus (LC) containing norepinephrine (NE). This pathway also receives contributions from peptidergic neurons in the lateral hypothalamus (LH) containing orexin (OX) or melanin-concentrating hormone (MCH), and from basal forebrain (BF) neurons that contain γ-aminobutyric acid (GABA) or ACh. Note that all of these ascending pathways traverse the region at the junction of the brainstem and forebrain where lesions cause profound sleepiness. (Reproduced with permission from Saper CB, Scammell TE, Lu J. Hypothalamic regulation of sleep and circadian rhythms. Nature. 2005;437(7063):1257–1263.)

The ARAS consists of several different circuits including the four main monoaminergic pathways discussed in Chapter 6. The norepinephrine pathway originates from the LC and related brainstem nuclei; the serotonergic neurons originate from the RN within the brainstem as well; the dopaminergic neurons originate in the ventral tegmental area (VTA); and the histaminergic pathway originates from neurons in the tuberomammillary nucleus (TMN) of the posterior hypothalamus. As discussed in Chapter 6, these neurons project widely throughout the brain from restricted collections of cell bodies. Norepinephrine, serotonin, dopamine, and histamine have complex modulatory functions and, in general, promote wakefulness. The PT in the brainstem is also an important component of the ARAS. Activity of PT cholinergic neurons (REM-on cells) promotes REM sleep, as noted earlier. During waking, REM-on cells are inhibited by a subset of ARAS norepinephrine and serotonin neurons called REM-off cells.
The VLPO area of the anterior hypothalamus consists mainly of inhibitory neurons that release γ-aminobutyric acid (GABA) and the neuropeptide galanin. The VLPO neurons are likely to have reciprocal interactions with the ARAS and orexin neurons. The VLPO neurons inhibit and are inhibited by the TMN histamine neurons and REM-off monoamine neurons. Orexin neurons are located in the lateral hypothalamus. They are organized in a widely projecting manner, much like the monoamines (Chapter 6), and innervate all of the components of the ARAS. They excite the REM-off monoaminergic neurons during wakefulness and the PT cholinergic neurons during REM sleep. They are inhibited by the VLPO neurons during NREM sleep.
According to a simplified model, the onset of NREM sleep is driven by VLPO area neurons that exert an inhibitory effect on TMN histamine neurons 13–3. Consistent with this hypothesis is the finding that VLPO and TMN neurons exhibit opposite patterns of activity during the sleep–wake cycle. In contrast to the VLPO neurons, which are active during sleep, the TMN neurons are persistently active during wakefulness, reduce their firing during NREM sleep, and become inactive during REM sleep. The role of the TMN histamine neurons in sleep–wake activity explains how the older antihistamines such as diphenhydramine andchlorpheniramine, which are H1 receptor antagonists (or inverse agonists), promote drowsiness and sleep. Newer antihistamines, such as loratadine, do not cross the blood–brain barrier and are without sedative effects. The older antihistamines are still marketed as over-the-counter sleep aids.
In the absence of tonic activity from the ARAS and TMN, which promotes wakefulness, higher-frequency oscillations in the cortex disappear, giving rise to the synchronous firing of a large thalamocortical neuronal ensemble. Thus, the delta wave and spindling rhythms observed during NREM sleep most likely reflect intrinsic properties of thalamic neurons, which synchronize the large thalamocortical network. Because a large number of neurons fire in synchrony at this stage, EEG recordings detect high-amplitude waves (see 13–1).
The brain structures involved in the control of NREM sleep are also crucial to thermoregulation. Some neurons in the preoptic area of the hypothalamus (POAH) are thermosensitive; they respond to changes in temperature by activating broad thermoregulatory mechanisms. In fact, as indicated previously, NREM sleep is associated with a lowering of the set point for body temperature, a loss of body heat, and a decline in energy metabolism. Warm-sensitive cells in the POAH area appear to be sleep-active, whereas cold-sensitive cells are active during wakefulness. Consistent with these observations, warm-sensitive POAH neurons increase their firing rates during the transition from wakefulness to NREM sleep, whereas cold-sensitive neurons reduce their firing rates during the onset of sleep. Furthermore, warming of the POAH induces NREM sleep, increases the length of NREM periods, and increases delta activity during slow-wave sleep. In contrast, POAH lesions result in chronic disruption of both NREM sleep and thermoregulation. It therefore appears that warm-sensitive neurons in the POAH regulate the onset and maintenance of slow-wave sleep. Interestingly, passive heating of the body improves sleep continuity in patients with insomnia.
In contrast to the diffuse brain structures involved in NREM sleep, the neuroanatomy of REM-active structures is relatively circumscribed. As previously mentioned, the primary oscillator that drives REM sleep appears to be located in the PT 13–3. REM-on cholinergic neurons in the PT, which includes the pedunculopontine tegmental (PPT) and laterodorsal tegmental (LDT) nuclei, have high discharge rates during wakefulness and REM sleep and low discharge rates during NREM sleep. During NREM sleep, the VLPO area neurons start inhibiting the orexin neurons of the lateral and posterior hypothalamus. Consequently, the norepinephrine and serotonin REM-off cells, which are excited by orexin neurons during wakefulness, start to wane in activity, which gradually releases the cholinergic REM-on cells from this inhibitory effect. At the end of NREM sleep, the VLPO area neurons directly inhibit the REM-off cells, which completely disinhibits the REM-on cholinergic neurons and initiates REM sleep. Consistent with the inhibition of REM-on cells by serotonergic and noradrenergic inputs, antidepressant drugs, which increase the availability of synaptic serotonin or norepinephrine (Chapter 15), reduce REM sleep. Older tricyclic antidepressants, which are also anticholinergic (ie, they inhibit muscarinic cholinergic receptors), likely reduce REM sleep by the latter mechanism as well.
Via their heavy projections to thalamic nuclei and the reticular formation, the REM-on cells result in EEG desynchronization. Not surprisingly, application of cholinergic agonists or acetylcholinesterase inhibitors to the target cells in the thalamic nuclei or the reticular formation activates REM sleep, whereas muscarinic cholinergic antagonists suppress REM sleep.
Activity in the PT is responsible not only for initiating REM sleep but also for the physical manifestations of REM sleep. For example, cholinergic projections from the PT to the medulla and spinal cord induce muscle atonia, most likely by hyperpolarizing motor neurons and associated interneurons. REM-associated cortical asynchrony, phasic eye movements, and cardiopulmonary accelerations and decelerations involve projections from the pons to the hypothalamus and thalamus. Although discrete pontine structures initiate most tonic and phasic elements of REM sleep, other structures are involved in REM regulation. For example, orexin neurons appear to be critical for the regulation of REM sleep, as discussed in the section “Narcolepsy.” In addition, inputs from the amygdala and other limbic structures can influence phasic REM activity. This finding may explain why stress and depression are associated with increased phasic REM activity. Furthermore, lesions caudal and rostral to pontine REM-on cells profoundly alter the characteristics of REM sleep; for instance, such lesions can cause REM sleep without atonia, which can lead to the motoric expression of dreams. Such motor activity in humans during sleep has been associated with unintentional nocturnal violence against sleeping partners and injury from falls.
Sleep is partly controlled by a homeostatic drive that increases the propensity to fall asleep in response to the amount of prior wakefulness. A student who has “pulled an all-nighter” may take an afternoon nap in response to a strong homeostatic drive resulting from an unmet need for sleep. This homeostatic drive is reflected by the amount of EEG slow-wave activity. However, the propensity to sleep is also affected by a circadian process 13–4. The student who stays awake to study may feel increasingly tired throughout the night, and yet may feel more alert at 8:00 AM than at 4:00 AM, despite the fact that by 8:00 AM 4 additional hours of sleep debt have accumulated.
13–4
A two-process model of sleep regulation. The graph indicates the time course of the homeostatic process that promotes sleep (S) and the circadian process (C) that influences wakefulness. Awakening occurs at the intersection of S and C. (Reproduced with permission from Borbély AA: A two process model of sleep regulation, Hum Neurobiol. 1982;1(3):195–204.)

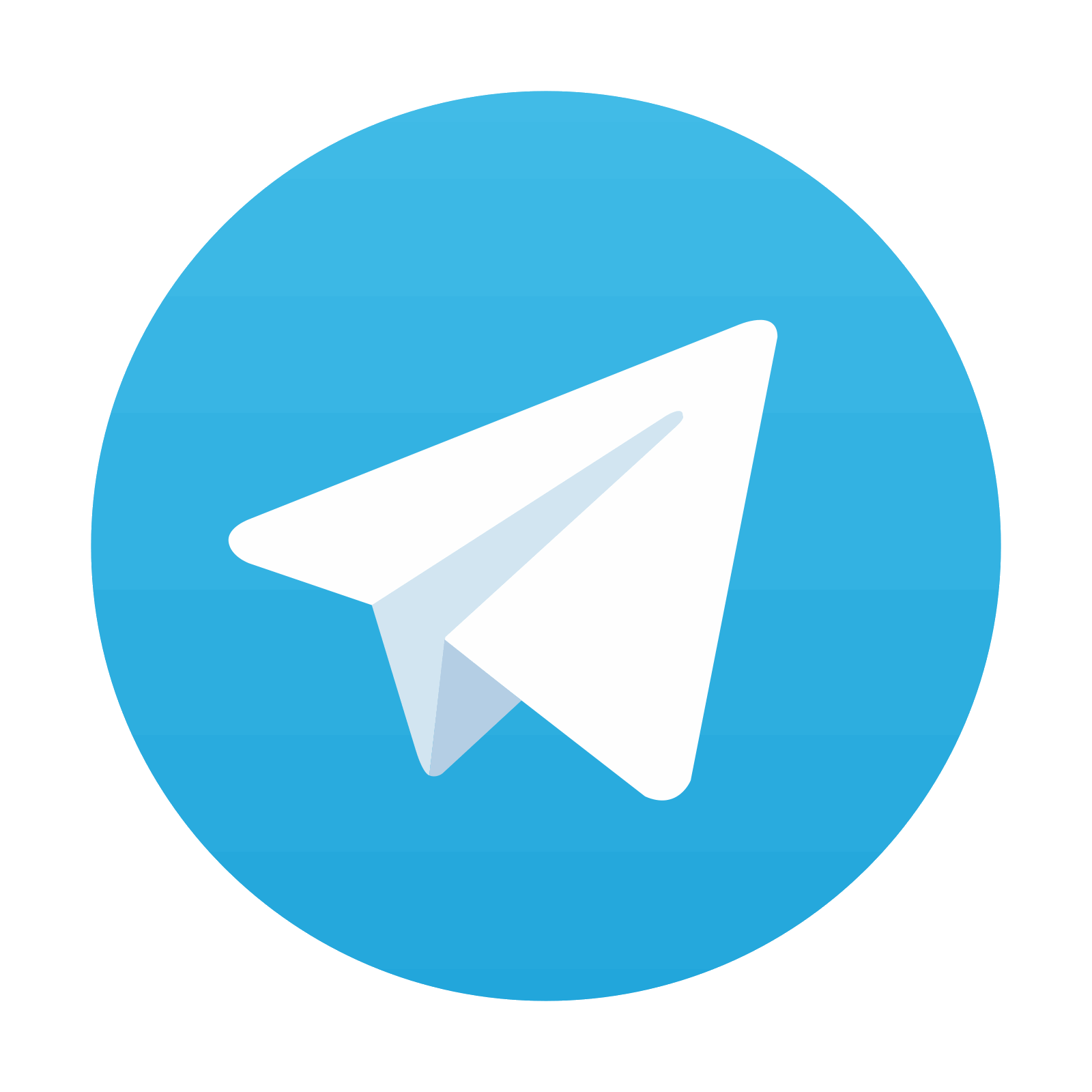
Stay updated, free articles. Join our Telegram channel
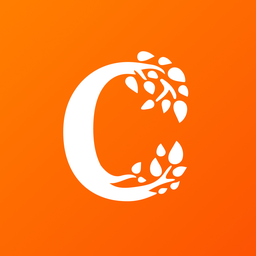
Full access? Get Clinical Tree
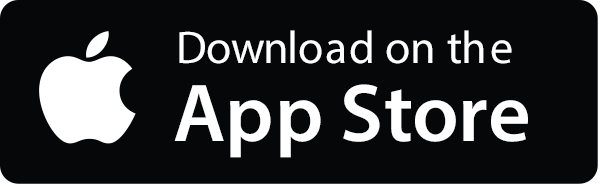
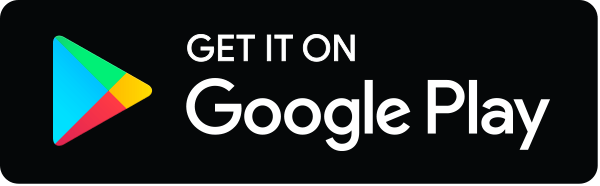
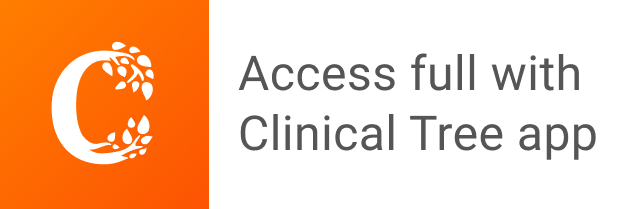