Chapter 10 Spinal Cord
The Spinal Cord Is Segmented
An adult human spinal cord appears surprisingly small on first inspection, being only about 42 to 45 cm long and about 1 cm in diameter at its widest point. It weighs only about 35 g, so one could be mailed for just two stamps. It is anatomically segmented—not obviously, like an earthworm, but in terms of the nerve roots attached to it (Fig. 10-1). A continuous series of dorsal (i.e., posterior) rootlets enter the cord in a shallow longitudinal groove (the posterolateral sulcus) on its posterolateral surface, and a continuous series of ventral (i.e., anterior) rootlets leaves from the poorly defined anterolateral sulcus. The dorsal and ventral rootlets from discrete sections of the cord coalesce to form dorsal and ventral roots, which in turn join to form spinal nerves (Fig. 10-2). Each dorsal root bears a dorsal root ganglion just proximal to the junction between dorsal and ventral roots; it contains the cell bodies of the primary sensory neurons whose processes travel through that particular spinal nerve. A portion of the cord that gives rise to a spinal nerve constitutes a segment. There are 31 segments in a human spinal cord: 8 cervical, 12 thoracic, 5 lumbar, 5 sacral, and 1 coccygeal.
The spinal cord itself, stripped of its dorsal and ventral rootlets, gives no obvious sign of segmentation. Rather, it is a continuous column with two enlargements that ends caudally in the pointed conus medullaris (Fig. 10-3). The two enlargements occur in those regions of the cord that supply the upper and lower extremities and therefore contain increased numbers of motor neurons and interneurons. The limits of the enlargements are not distinct, but the cervical enlargement, which supplies the upper extremities, is conventionally considered to extend from the fifth cervical to the first thoracic segment (C5 to T1), inclusive. The lumbar (or lumbosacral) enlargement, which supplies the lower extremities, extends from the second lumbar to the third sacral segment (L2 to S3).
Each Spinal Cord Segment Innervates a Dermatome
As the neural tube closes, adjacent mesoderm also segments, here into a series of somites (see Fig. 2-3) that will give rise to skin, muscle, and bone. Each spinal nerve retains its relationship with a somite during development, with the result that spinal cord segments are related systematically to areas of skin, to muscles, and in some instances to bones (e.g., vertebrae). Hence each spinal nerve (except C1, which typically has only a rudimentary dorsal root) innervates a single dermatome (Fig. 10-4). This dermatomal arrangement is particularly apparent in the trunk, where pairs of dermatomes form bands that encircle the chest and abdomen; outgrowth of limb buds during development makes the dermatomal arrangement somewhat more complex in the upper and lower extremities. * Similarly, the innervation of skeletal muscles is related systematically to spinal segments (Table 10-1).
Table 10-1 Innervation of Major Muscles
Movement | Peripheral Nerve (Muscle) | Cord Segment* |
---|---|---|
Arm | ||
Abduction | Suprascapular (supraspinatus) | C5, C6 |
Axillary (deltoid) | C5, C6 | |
Elbow | ||
Flexion | Musculocutaneous (brachialis, biceps) | C5, C6 |
Radial (brachioradialis) | C5, C6 | |
Extension | Radial (triceps) | C6, C7, C8 |
Wrist | ||
Flexion | Median, ulnar | C6, C7, C8 |
Extension | Radial | C5, C6, C7, C8 |
Hand | ||
Finger movements | Median, radial, ulnar | C7, C8, T1 |
Thumb movements | Median, radial, ulnar | C7, C8, T1 |
Hip | ||
Flexion | Lumbar spinal nerves, femoral (iliopsoas) | L1, L2, L3 |
Extension | Inferior gluteal (gluteus maximus) | L5, S1, S2 |
Knee | ||
Flexion | Sciatic (hamstrings) | L5, S1, S2 |
Extension | Femoral (quadriceps) | L2, L3, L4 |
Ankle | ||
Dorsiflexion | Sciatic → peroneal (tibialis anterior) | L4, L5 |
Plantar flexion | Sciatic → tibial (gastrocnemius) | S1, S2 |
* Major segments indicated in bold.
Knowledge of the segmental innervation of muscles and cutaneous areas (Table 10-2) can be extremely helpful in diagnosing the site of damage in or near the spinal cord. For example, compression of a dorsal root can cause pain in its dermatome, allowing pain caused by root compression to be differentiated from pain caused by peripheral nerve damage. In addition, the highest level of a sensory or motor deficit may allow deductions about the segmental level of a suspected spinal cord lesion (see Fig. 10-31).
Table 10-2 Dermatomal Levels of Clinical Importance*
Cutaneous Area | Cord Segment |
---|---|
Upper arm (lateral surface) | C5 |
Thumb and lateral forearm | C6 |
Middle finger | C7 |
Little finger | C8 |
Nipple | T4 |
Umbilicus | T10 |
Big toe | L5 |
Heel | S1 |
Back of the thigh | S2 |
* See Figure 10-4 for additional details.
The Spinal Cord Is Shorter Than the Vertebral Canal
The spinal cord approaches its adult length before the vertebral canal does. Until the third month of fetal life, both grow at about the same rate, and the cord fills the canal. Thereafter the body and the vertebral column grow faster than the spinal cord does, so that at the time of birth the spinal cord ends at the third lumbar vertebra. A small additional amount of differential growth in the vertebral column occurs subsequent to this, and by a few months of age the cord ends at about the level of the first lumbar vertebra. However, the spinal nerves still exit through the same intervertebral foramina as they did early in development, and each dorsal root ganglion remains at the level of the appropriate foramen. Proceeding from cervical to sacral levels, the dorsal and ventral roots become progressively longer because they have longer and longer distances to travel before reaching their sites of exit from the vertebral canal (Fig. 10-3A). The lumbar cistern, from the end of the spinal cord at vertebral level L1-L2 to the end of the dural sheath at vertebral level S2, is filled with this collection of dorsal and ventral roots, collectively referred to as the cauda equina (Latin for “horse’s tail”; Fig. 10-5E and F). Hence a needle carefully inserted into the lumbar cistern will pass harmlessly among nerve roots, allowing safe sampling of cerebrospinal fluid (CSF).
The meningeal coverings of the spinal cord were described in Chapter 4 (see Fig. 4-13). The cord is suspended within an arachnoid-lined dural tube by the denticulate ligaments (Fig. 10-6A), which are extensions of the pia-arachnoid, similar to but more substantial than arachnoid trabeculae. In addition, the caudal end of the cord is anchored to the end of the dural tube by the filum terminale (Fig. 10-6B), an extension of the pial covering of the conus medullaris. The filum terminale then acquires a dural outer layer and in turn is anchored to the coccyx.
All Levels of the Spinal Cord Have a Similar Cross-Sectional Structure
In cross section the spinal cord consists of a roughly H-shaped area of gray matter that floats like a butterfly in a surround of white matter. The gray matter can be divided into horns and the white matter into funiculi (from the Latin funiculus, meaning “string”) (Fig. 10-7). Keep in mind that the spinal cord is, to a great extent, a longitudinally organized structure, even though it is most conveniently studied in cross section. For example, the posterior gray horns are continuous cell columns rather than a series of discrete nuclei, and at any given level the posterior horn cells interact with cells from many other levels.
In addition to the posterolateral and anterolateral sulci, several other longitudinal grooves indent the cross-sectional outline of the cord (Fig. 10-7). The deep anterior median fissure extends almost to the center of the cord; at the apex of this fissure, only a thin zone of white matter (the anterior white commissure*) and a thin zone of gray matter separate the central canal from subarachnoid space. The posterior median sulcus is much less distinct, but a glial septum extends from it all the way to the gray matter surrounding the central canal. Therefore the two sides of the spinal cord can communicate with each other only through a narrow band of neural tissue near the central canal. Because the fibers of some ascending pathways cross the midline in the spinal cord, this small area where crossing occurs may be clinically important in diseases affecting the center of the cord (see Fig. 10-32). Finally, at cervical and upper thoracic levels, a posterior intermediate sulcus is found. Another glial septum projects from this sulcus, partially subdividing each posterior funiculus.
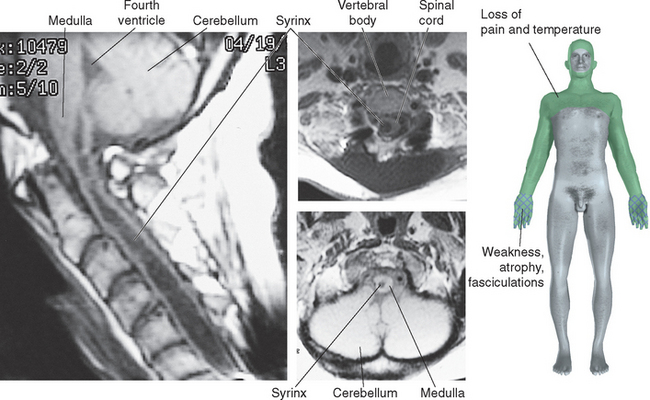
Figure 10-32 Syringomyelia. A 39-year-old man was thrown from the bed of a pickup truck in a motor vehicle accident, sustaining vertebral fractures at T5-T6 and C4-C5. About 2 years later he began to notice progressive weakness and atrophy of his hand muscles. Magnetic resonance imaging revealed a central cavity in his spinal cord (syringomyelia), extending into the caudal medulla. The cavity is somewhat irregular in shape, and damage is more extensive at some levels than at others. Typical findings in such a case would be a band of bilateral loss of pain and temperature sensation (extending into the distribution of the trigeminal nerve, in a pattern explained in Chapter 12) and weakness and atrophy at levels where the damage extends into the anterior horns (in this case, at lower cervical levels).
(Magnetic resonance images, courtesy Dr. Raymond F. Carmody, University of Arizona College of Medicine.)
The Spinal Cord Is Involved in Sensory Processing, Motor Outflow, and Reflexes
Afferent fibers enter the cord via the dorsal roots* and then end almost exclusively on the ipsilateral side of the CNS. They may reach their site of termination either by synapsing on neurons in the ipsilateral gray matter of the spinal cord or by ascending directly and uncrossed to relay nuclei in the medulla. The relay cells in the spinal gray matter or the medulla then project their axons through defined sensory pathways to more rostral structures. In subsequent discussions of these sensory pathways, it may sometimes sound as if a particular primary afferent synapses on only one relay cell and sends its information into only one pathway. However, it is important to realize that each primary afferent fiber gives rise to many branches and feeds into more than one ascending sensory pathway and into local reflex circuits as well (see Fig. 3-27). It is estimated, for example, that a single Ia afferent from a muscle spindle may give rise to 500 or more branches within the spinal cord.
Spinal Gray Matter Is Regionally Specialized
The Posterior Horn Contains Sensory Interneurons and Projection Neurons
The posterior horn consists mainly of interneurons whose processes remain within the spinal cord and of projection neurons whose axons collect into long, ascending sensory pathways. This area of gray matter contains two prominent parts, the substantia gelatinosa and the body of the posterior horn, both present at all spinal levels.
The substantia gelatinosa is a distinctive region of gray matter that caps the posterior horn (Fig. 10-8). In myelin-stained preparations this region looks pale compared with the rest of the gray matter because it deals mostly with finely myelinated and unmyelinated sensory fibers that carry pain and temperature information. Between the substantia gelatinosa and the surface of the cord is a relatively pale-staining area of white matter called Lissauer’s tract. * This tract stains more lightly than the rest of the white matter because it contains the finely myelinated and unmyelinated fibers with which the substantia gelatinosa deals.
The Anterior Horn Contains Motor Neurons
The anterior horn contains the cell bodies of the large motor neurons that supply skeletal muscle (Fig. 10-9). These alpha motor neurons, also referred to as lower motor neurons, * are the only means by which the nervous system can exercise control over body movements, whether voluntary or involuntary; a number of different parts and pathways of the nervous system can influence these lower motor neurons, but they alone can elicit muscle contraction. Destruction of the lower motor neurons supplying a muscle or interruption of their axons therefore causes complete paralysis of that muscle. Lower motor neuron lesions cause paralysis of a type called flaccid paralysis, indicating that the muscle is limp and uncontracted. Reflex contractions can no longer be elicited, and the muscle slowly atrophies (owing to a lack of trophic factors normally delivered to it by motor axons; see Chapter 24). This occurs, for example, in poliomyelitis (a viral disease that attacks the motor neurons of the anterior horn) and in injuries in which ventral roots are damaged.
Alpha motor neurons occur in longitudinally oriented, cigar-shaped groups, each group innervating an individual muscle. Hence in cross sections they appear to be arranged in clusters (Fig. 10-10), separated from one another by areas of interneurons; the clusters that innervate axial muscles are medial to those that innervate limb muscles. In the cervical and lumbar enlargements, which innervate the limbs, the anterior horns are enlarged laterally to accommodate the additional motor neurons (Fig. 10-8). Smaller gamma motor neurons are interspersed with alpha motor neurons in all such groups. They innervate the intrafusal muscle fibers of muscle spindles, so they are also referred to as fusimotor neurons.
Two columns of motor neurons in the anterior horn of the cervical cord are recognized as separate entities. The spinal accessory nucleus extends from the caudal medulla to about C5. The axons of these motor neurons emerge from the lateral surface of the spinal cord just posterior to the denticulate ligament as a separate series of rootlets that form the accessory nerve (see Fig. 3-17). The phrenic nucleus, containing the motor neurons that innervate the diaphragm, is located in the medial portion of the anterior horn in segments C3 to C5. This makes injuries to the upper cervical spinal cord a matter of grave concern, because destruction of the descending pathways that control the phrenic nucleus and other respiratory motor neurons renders a patient unable to breathe.
The Intermediate Gray Matter Contains Autonomic Neurons
The preganglionic sympathetic neurons for the entire body lie in segments T1 through L3, most of them located in a column of cells called the intermediolateral cell column, which forms a pointy lateral horn on the spinal gray matter (Fig. 10-8). Their axons leave through the ventral roots. Cells in a corresponding location in segments S2 to S4 constitute the sacral parasympathetic nucleus but do not form a distinct lateral horn. Their axons leave through the ventral roots and synapse on the postganglionic parasympathetic neurons for the pelvic viscera.
Clarke’s nucleus (or the nucleus dorsalis) is a rounded collection of large cells located on the medial surface of the base of the posterior horn from about T1 to L2. It is particularly prominent at lower thoracic levels (Fig. 10-8). This is an important relay nucleus for the transmission of information to the cerebellum and may also play a role in forwarding proprioceptive information from the leg to the thalamus. Because of its prominent role in sensory processing, it is treated by many as part of the posterior horn.
Spinal Cord Gray Matter Is Arranged in Layers
In 1952 Rexed devised a system for subdividing the gray matter of the cat’s spinal cord into layers, or laminae. The same system has since been applied to the cords of other mammals, including humans (Fig. 10-7B). Lamina I (also called the marginal zone) is a thin layer of gray matter that covers the substantia gelatinosa, lamina II is the substantia gelatinosa, and laminae III through VI are the body of the posterior horn; lamina VII roughly corresponds to the intermediate gray matter (including Clarke’s nucleus) but also includes large extensions into the anterior horn; lamina VIII comprises some of the interneuronal zones of the anterior horn, whereas lamina IX consists of the clusters of motor neurons embedded in the anterior horn; lamina X is the zone of gray matter surrounding the central canal.
This terminology has proved useful for experimental anatomists and physiologists because the histological differences among the laminae correspond to functional differences (Table 10-3). For example, the functional dichotomy between large- and small-diameter peripheral nerve fibers is maintained to a great extent in the patterns of termination of these fibers in the spinal gray matter: there are prominent (though not exclusive) terminations of pain and temperature afferents in laminae I and II, tactile afferents from cutaneous nerves in lamina III, and Ia muscle spindle afferents in laminae VI, VII, and IX.
Reflex Circuitry Is Built into the Spinal Cord
A reflex is an involuntary, stereotyped response to a sensory input. All reflex pathways, other than axon reflexes (see Fig. 9-13), therefore must involve at least a receptor structure and associated afferent neuron (with its cell body in a dorsal root ganglion or some other sensory ganglion) and an efferent neuron (with its cell body within the CNS). With the exception of the stretch reflex, all reflexes involve one or more interneurons as well.
Muscle Stretch Leads to Excitation of Motor Neurons
All skeletal muscles (except perhaps some muscles in the head) contract to at least some extent in response to being stretched. The reflex arc responsible for this contraction utilizes the simplest possible route through the CNS because it involves only two neurons and a single intervening synapse. It is therefore sometimes referred to as the monosynaptic reflex or the myotatic reflex (from two Greek words meaning “muscle stretch”). The afferent limb of the arc is a Ia afferent with its associated muscle spindle primary ending. Central processes of the Ia afferent make synapses within the spinal cord directly on the alpha motor neurons that innervate the muscle containing the stimulated spindle (Fig. 10-11).
The stretch reflex is commonly used for clinical testing purposes. Tapping the patellar tendon, as in the familiar knee-jerk reflex, stretches the quadriceps slightly. Ia endings in quadriceps muscle spindles are excited and in turn excite quadriceps alpha motor neurons; these cause the quadriceps to contract, completing the reflex. Similarly, tapping the Achilles tendon stretches the gastrocnemius slightly, thereby causing a reflex contraction. Testing a variety of stretch reflexes can provide valuable clinical information about the integrity not only of peripheral nerves but also of predictable spinal cord segments (Table 10-4). Because stretch reflexes are usually elicited by tapping a tendon, they are often referred to as deep tendon reflexes (sometimes abbreviated as DTRs). One should remember that even though the reflex is studied in this manner, the responsible receptors are actually in the muscles attached to the tapped tendons.
Stretch reflexes are thought to be important for the constant automatic corrections we perform during movements and postures (although other reflexes may in fact be even more important for this function). As an example, when we stand still and upright, we actually sway to and fro a bit. Each time we sway in one direction, some muscles are stretched, and the resulting reflex contraction helps return us toward the desired position.
Muscle Tension Can Lead to Inhibition of Motor Neurons
Stimulation of a Ib fiber from a Golgi tendon organ has an effect that varies, depending on the position and activity of the limb at the time of stimulation. It sometimes has an effect opposite to that of stimulating a Ia fiber: the alpha motor neurons that innervate the muscle connected to that tendon organ are inhibited. This effect is a form of autogenic inhibition and involves an inhibitory interneuron between the afferent and efferent fibers (Fig. 10-12). Under other circumstances (e.g., stimulating a tendon organ attached to a weight-supporting muscle), excitation of the motor neurons can result (again, through an interneuron).
Painful Stimuli Elicit Coordinated Withdrawal Reflexes
The flexor reflex pathways in the spinal cord are normally held in a somewhat inhibited state by descending influences from the brainstem, so that only noxious stimuli result in a strong reflex. If these descending influences are removed, either surgically in experimental animals or as a result of some pathological condition, reflex flexion can result from harmless tactile stimulation. This indicates that most or all cutaneous receptors feed into the pathway, but ordinarily only nociceptors have a powerful enough influence to cause a reflex withdrawal.
Because the flexor reflex involves an entire limb, its pathway must spread over several spinal segments to include the motor neurons innervating all the various flexor muscles of that limb. This spreading occurs in two ways. First, all primary afferent fibers bifurcate on entering the spinal cord, and their processes then extend one or more segments in both rostral and caudal directions. Second, the flexor pathway includes at least one interneuron, which itself may have processes extending over several segments (Fig. 10-13).
Reflexes Are Accompanied by Reciprocal and Crossed Effects
It would clearly be easier to shorten a stretched muscle if the motor neurons to its synergists were excited and those to its antagonists inhibited. This reciprocal inhibition actually does occur and is a general principle in all reflexes: reflex activity in a given muscle produces similar activity in its ipsilateral synergists and the opposite activity in its ipsilateral antagonists (Fig. 10-14). Thus the standard tap on the patellar tendon causes not only excitation of quadriceps motor neurons but also inhibition (through an interneuron) of motor neurons to the hamstring muscles. If one extensor muscle of the thigh were selectively stretched, its motor neurons would be monosynaptically excited, as would those of all the other thigh extensors. After stimulation of a Golgi tendon organ the pattern may be just the reverse: if tension is applied to the patellar tendon during certain phases of a movement, the quadriceps is inhibited and the hamstring muscles are excited, both actions occurring through interneurons. Finally, the flexor reflex is accompanied by inhibition of the extensors of that limb.
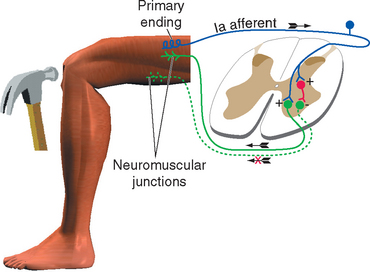
Figure 10-14 Reciprocal inhibition. Striking the patellar tendon initiates a stretch reflex, as in Figure 10-11. It also causes inhibition, through an interneuron, of the motor neurons to the antagonist hamstring muscles.
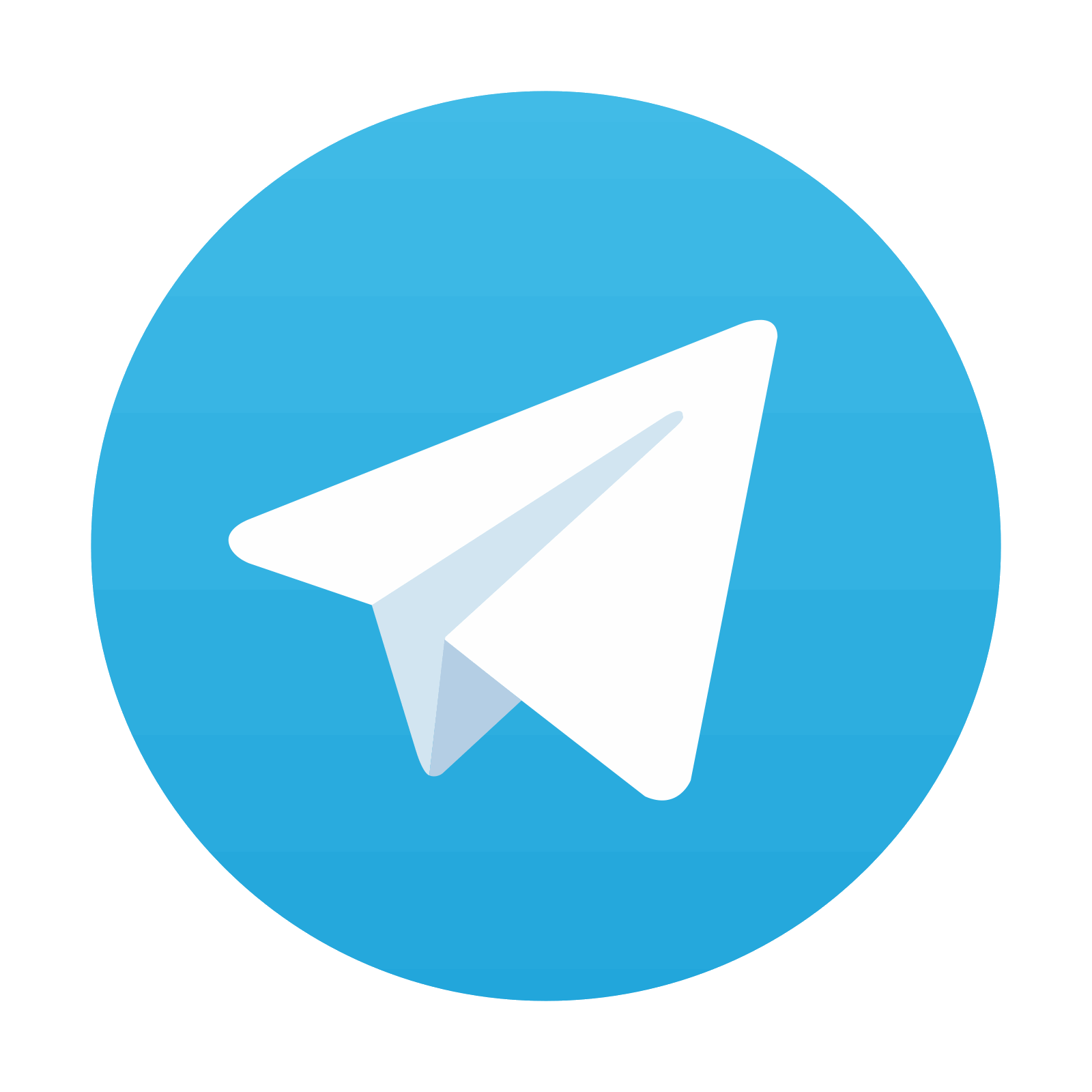
Stay updated, free articles. Join our Telegram channel
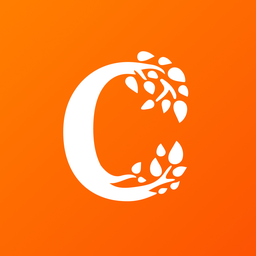
Full access? Get Clinical Tree
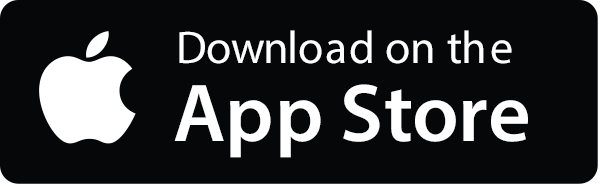
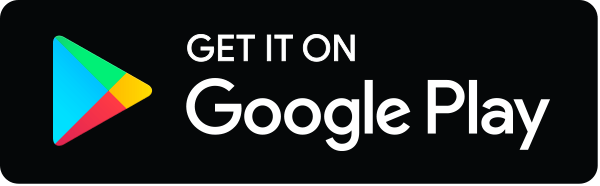