Abstract
Spinal cord stimulation (SCS) has been used in managing chronic pain conditions that are refractory to current pharmacotherapies. However, conventional SCS has often been associated with suboptimal efficacy and short-lived therapeutic effects. It is important to further improve the clinical efficacy of SCS by enhancing our understanding of its mechanisms of action (MOAs). Here, we review research on MOA in the past decade (2006–2016) and highlight important findings regarding the biological basis by which different paradigms of SCS may produce pain inhibition. In addition, we discuss future research directions. The potential mechanisms and neuronal substrates that may underlie the paresthesia-free pain inhibition by the novel kilohertz high-frequency SCS are further reviewed in more detail. Additionally, we describe the recently developed methods and rationales of recording electrically evoked compound action potential as an in situ electrophysiological measure to provide diagnostic and prognostic indications for chronic pain sufferers and to evaluate SCS.
Keywords
Compound action potential, Frequency, Mechanisms of action, Nerve injury, Neuron, Neurostimulation, Pain, Spinal cord
Outline
Introduction 162
Historical Review and Current Technology 162
Conventional Spinal Cord Stimulation 162
High-Frequency Spinal Cord Stimulation 162
Burst Spinal Cord Stimulation 162
Spinal Nociceptive Transmission 162
Mechanisms of Action Overview 163
Activation of the Dorsal Column 163
Suppression of Dorsal Horn Neuronal Activity and Sensitization 164
Conventional Tonic Spinal Cord Stimulation 164
High-Frequency Spinal Cord Stimulation 164
Burst Spinal Cord Stimulation 165
Modulation of Spinal Pain Microcircuitry 165
Supraspinal Mechanisms 165
Stimulation Parameters 166
Neurochemical Mechanisms 166
High-Frequency Spinal Cord Stimulation 167
Use of the Electrically Evoked Compound AP to Study Types of Fibers Activated by SCS 169
Future Directions 173
Conclusions 173
References 174
Introduction
Historical Review and Current Technology
Conventional Spinal Cord Stimulation
Treatment of chronic pain remains a large unmet medical need. The seminal “gate control” theory of pain postulates that stimulation of low-threshold afferents (Aβ-fibers) would result in an inhibition of spinal nociceptive transmission ( ). This theory led to the development of viable pain therapies including conventional spinal cord stimulation (SCS) and transcutaneous electrical nerve stimulation (TENS) ( ). A contemporary view of gate control now includes the broadened “neuromatrix” concept that encompasses multiple interacting neuronal circuits ( ). Conventional SCS is produced by delivering mild electrical pulses through electrodes placed in the epidural space overlaying the dorsal columns (DCs), typically at an intensity that elicits paresthesia, at a frequency of 30–60 Hz.
SCS has been shown to provide pain relief and improve quality of life in patients with refractory pain ( ), the most common indications having been neuropathic pain of peripheral origin or “mixed pain conditions” with a dominant neuropathic component such as complex regional pain syndrome (CRPS) and failed back surgery syndrome (FBSS) ( ). Conventional SCS remains the mainstay and most used neurostimulation pain therapy ( ), and has, in many published studies, performed well in comparison with other therapies including “conventional medical management” (CMM) when investigating safety, appropriateness, fiscal neutrality, and effectiveness, the SAFE principles recently introduced into relevant literature by . Based on the SAFE principles, systematic analysis of clinical evidence suggested that SCS should be considered before submitting a patient to either long-term systemic opioid therapy or repeat spinal surgery for chronic pain resulting from FBSS and CRPS but not as a last resort in pain therapeutic algorithms ( ).
High-Frequency Spinal Cord Stimulation
Recently, a new, high-frequency SCS (HF-SCS, e.g., 10 kHz) paradigm has been introduced for pain treatment that is paresthesia free ( ). Like conventional SCS, HF-SCS (1 and 10 kHz) inhibits mechanical hypersensitivity in an intensity-dependent fashion in a rat model of neuropathic pain, and certain types of HF-SCS may have a greater effect or require a lower intensity for pain inhibition than conventional SCS ( ). A randomized and controlled clinical trial also suggests that subliminal/paresthesia-free HF-SCS may be superior to conventional SCS in the long-term treatment of back and leg pain ( ).
Burst Spinal Cord Stimulation
In the 1970s, burst TENS was launched as a variant to tonic TENS with very low, acupuncture-like frequency or normal TENS at about 100 Hz ( ). Hypotheses about different mechanisms for burst and high-frequency TENS were also discussed at that time, with burst stimulation seen as more appropriate for inhibiting nociceptive pain involving a release of endogenous opioids. The concepts regarding burst SCS (bursts of five pulses with internal frequency of 500- and 40-Hz interburst frequency), introduced by De Ridder et al., stems from his work with cortical stimulation ( ). In several clinical studies, burst SCS, administered with subsensory amplitudes, was initially presented as a stimulation mode that was more effective than conventional SCS for the lumbosacral pain and nociceptive back pain component of FBSS ( ). It is reported that 63% of nonresponders to conventional SCS respond to burst stimulation. In addition, 95% of the responders to tonic SCS experienced further improvement with burst stimulation ( ). Thus, burst SCS may significantly rescue nonresponders to conventional SCS and further improve pain inhibition in responders to conventional SCS. Recent studies suggest that burst SCS may also be more efficacious than tonic 50-Hz SCS in inhibition of neuropathic pain and visceral nociception and the improvement of physical activity ( ). Nevertheless, a recent systematic review suggests that the clinical evidence for burst SCS, in managing chronic intractable pain, remains insufficient ( ).
Spinal Nociceptive Transmission
Mammalian, primary, afferent neurons of the dorsal root ganglia (DRG) and trigeminal ganglia are pseudo-unipolar, with central processes that terminate in the spinal cord dorsal horn (DH). Large-diameter, afferent neurons (Aβ) are mostly activated by innocuous mechanical stimuli such as skin stroking, indentation, and vibration ( ). These neurons form synaptic contacts onto intrinsic, DH neurons and postsynaptic DC (PSDC) neurons; many also send collateral, axonal branches via the DCs that terminate in the DC nuclei. Nociceptive, afferent neurons (C, Aδ) transmit noxious information to the spinal cord, principally to superficial lamina (I/II) and deep lamina (V) ( ). Sensory information can be integrated and modified at the central terminals of afferent fibers and at the synaptic junctions of DH neurons, before their dispatch to supraspinal centers. Thus, the DH serves as a relay station and an important site for the integration and modulation of converging noxious and innocuous information ( Fig. 15.1A ).

Wide-dynamic range (WDR) neurons and nociceptive specific neurons (NSs) in the DH receive high-threshold/nociceptive afferent inputs (Aδ/C-fibers) ( ). WDR neurons also receive converging inputs from low-threshold/non-nociceptive Aβ-fibers and are the “transmission” cells of the gate control theory ( ). More WDR neurons are located in deeper than in superficial laminae of the DH, whereas NS neurons are found mostly in the superficial DH.
There are two pain pathways to supraspinal centers. The medial pain pathway encodes the motivational/affective component of pain, such as aversion and unpleasantness, whereas the lateral pathway mediates the sensory, discriminatory component of pain and projects to the ventral-posterolateral nuclei of the thalamus. The specific roles of WDR and NS neurons in the two pain pathways remain unclear, but WDR neurons are important for encoding the location and intensity of noxious stimuli, whereas NS neurons may transmit information that elicits the affective component of pain and triggers descending, pain modulation ( ).
Mechanisms of Action Overview
Activation of the Dorsal Column
Nerve lesion studies have shown that a large portion of pain inhibition produced by conventional SCS is through activation of the DCs, which contains axons of large-diameter, primary, afferent neurons and (deep in the DCs) the axons from PSDC neurons ( ). The gracile nucleus (GN) receives tactile information and is robustly activated in rats by conventional SCS at intensities that inhibit mechanical hypersensitivity ( ). However, pain inhibition by lower-intensity HF-SCS that may mimic clinical paresthesia-free HF-SCS does not activate the GN in neuropathic rats ( ). Furthermore, GN activity, evoked by peripheral, mechanical stimulation, is largely intact during HF-SCS. Thus, subliminal HF-SCS may not activate or change the conduction properties of DC fibers. These findings are consistent with computer models showing that clinical, paresthesia-free HF-SCS may not function through direct activation or conduction block of DC or DR fibers ( ). Furthermore, a recent study by shows that there is no activation of DC fibers to burst SCS, as measured by recording activity in the GN in the brainstem ( ).
Suppression of Dorsal Horn Neuronal Activity and Sensitization
Conventional Tonic Spinal Cord Stimulation
Pain inhibition by conventional SCS is intricately, though not exclusively, linked with suppression of DH neuronal activity ( ). Electrophysiological studies have revealed important features of neuronal inhibition by SCS that may correlate with clinical pain inhibition. Conventional SCS, using either surface ball electrodes or bipolar, needle electrodes inserted into the DCs of rats, decreases WDR neuronal activity ( ). SCS, in rats after nerve injury, inhibits spontaneous discharges and evoked responses to peripheral mechanical and electrical stimuli in WDR neurons ( ). These cellular mechanisms ( Fig. 15.1A ) may contribute to conventional SCS’s inhibition of ongoing pain and tactile allodynia ( ), two characteristic features of neuropathic pain ( ).
There is dynamic change of spinal neuronal circuits in response to different, environmental cues. Windup and long-term potentiation (LTP) in DH neurons may share certain mechanisms with central sensitization that underlies persistent pain ( ). Conventional SCS blocks windup ( ) and normalizes LTP in WDR neurons ( ). These physiologic data and computer model simulations suggest that inhibition of neuronal sensitization may be an important mechanism underlying neuropathic pain inhibition by SCS in humans ( ). A windup-like phenomenon, as from repetitive heat stimuli and repeated noxious mechanical stimuli, also occurs in humans during natural stimulation of C-fibers ( ).
It has been difficult to find predictors of conventional SCS analgesia in patients ( ). Recently, inhibition of the temporal summation of pain (e.g., windup) during trial SCS was shown to be helpful when selecting patients for the therapy and predicting the long-term efficacy of SCS ( ). This work presents a good example of how preclinical findings provide a conceptual framework and important rationale for improving clinical applications of SCS. Intriguingly, when used earlier after nerve injury (1 day), SCS produces more responders and longer pain reduction than when given later after nerve injury (16 days) ( ). This suggests that earlier intervention for neuronal sensitization with SCS may be more beneficial than when the therapy is introduced at a later period of time. Other spinal mechanisms may also underlie conventional SCS pain inhibition and include activation of descending pain-inhibitory pathways, induction of inhibitory postsynaptic potentials in DH neurons ( ), and/or facilitation of primary afferent depolarization, which elicits presynaptic inhibition of incoming afferent inputs ( ).
High-Frequency Spinal Cord Stimulation
Despite increasing clinical use, the neurophysiologic basis for pain inhibition by HF-SCS remains unclear. Using the antidromic, sciatic, compound action potential as a way to titrate stimulation intensity, investigators recently have shown that 50-Hz SCS inhibits WDR neurons in an intensity-dependent fashion ( ). At the high stimulation intensity that maximally activates Aβ-fibers in the DC (suprasensory threshold), and without activating Aδ/C-fibers, 1-kHz HF-SCS was less effective than 50-Hz SCS in suppressing windup, a short-term neuronal sensitization to repetitive noxious inputs, in WDR neurons ( ). This suggests that pain inhibition from kilohertz levels of frequency and 50-Hz SCS at suprathreshold intensities may involve different, spinal, neuronal mechanisms. Although, an earlier study suggests that 10-kHz HF simulation of the DR or at the dorsal root entry zone (DREZ) may depress hyperactivity in WDR neurons after nerve injury ( ), the stimulation site and intensity used in this study may not mimic those of clinical HF-SCS.
The onset of pain relief is often quick (minutes) following the introduction of conventional SCS in both patients and animal models ( ), but it takes much longer, sometime days, before pain inhibition occurs after paresthesia-free HF-SCS ( ). Accordingly, an important aspect to consider when studying the MOA differences between conventional and paresthesia-free HF-SCS may be the length of the stimulation period. Examining the neurophysiologic and neurochemical changes that may occur slowly and progressively at spinal levels after a longer period, subliminal HF-SCS may reveal new knowledge about its MOA.
Burst Spinal Cord Stimulation
Burst SCS attenuates DH, neuronal hyperactivity in a rat model of cervical radiculopathy ( ), and the attenuation of responses to noxious, somatic stimuli from burst stimulation is greater than attenuation to visceral stimuli in lumbosacral neurons ( ). Burst stimulation was thought to be more effective than tonic stimulation, because less temporal integration is needed to activate neurons, making it more effective as a “wake-up call to the brain.” An earlier functional magnetic resonance imaging study showed that conventional SCS predominantly modulates the lateral pain pathways and inhibits the somatosensory component of pain, as judged by changes in blood oxygen levels in the somatosensory cortices ( ). Burst SCS, comparatively, may primarily modulate the medial pain pathways and alleviate the affective component of pain ( ). Another hypothesis presented here relates, in a way, to an HF-SCS hypothesis: burst stimulation disrupts synchronous burst firing of high-threshold fibers, thus inhibiting an activation directly related to pain perception.
Modulation of Spinal Pain Microcircuitry
Central terminals of nociceptive afferent neurons mostly terminate in superficial DH, and different subpopulations of inhibitory interneurons (e.g., γ-aminobutyric acid [GABA]ergic) are differently distributed in superficial versus deeper lamina. Accordingly, the intrinsic network mechanisms by which SCS modulates superficial neurons may be different from that to modulate deep DH neurons ( ). So far, the effects of different SCS paradigms on NS neurons and superficial DH neurons remain largely unclear ( ). The substantia gelatinosa (SG, lamina II) of the DH consists of excitatory and inhibitory interneurons that form an important local pain regulatory circuitry ( ). A leading mechanistic theory of conventional SCS is that activity in Aβ-fibers attenuates pain transmission by activating spinal inhibitory interneurons ( ). A complementary morphologic study and electrophysiologic recording of evoked excitatory postsynaptic currents (eEPSCs) suggest that a subset of GABAergic interneurons are activated by Aβ-fiber inputs ( ) and may block spinal transmission of nociceptive inputs in Aδ/C-fibers ( ). Importantly, a brief train of 50- and 1-kHz electrical stimulation activates Aβ-fibers, inducing a sustained depression of eEPSCs to C-fiber inputs in SG neurons ( ). Yet, this novel form of heterosynaptic depression induced by Aβ-fiber stimulation occurred in both glutamatergic excitatory and GABAergic inhibitory neurons. Hence, conventional SCS may not induce excitatory neuron-selective inhibition as postulated by the gate control theory. The decreased excitability in GABAergic neurons may disinhibit pain transmission and compromise SCS analgesia, which may partially explain the short-lived, carryover inhibition after SCS ( ). Despite the fact that new evidence (also see details in HF-SCS section) of the MOA of other paradigms (e.g., burst SCS, HF-SCS) is emerging, future studies must examine whether these paradigms of SCS preferentially inhibit excitatory neurons and determine the net effect of SCS on projection neurons ( ).
Supraspinal Mechanisms
Conventional SCS activates the anterior pretectal nucleus of the brain, whose output activates major descending pain inhibitory pathways ( ), which, in turn, may increase c- fos expression in the brainstem pain modulatory circuitry ( ). A recent study suggests that up to 50% of the pain inhibitory effects from conventional SCS may be due to activation of supraspinal circuitry ( ). Both the rostral ventromedial medulla (RVM) and the locus coeruleus (LC) that make up the “spinal–brainstem–spinal loop” were activated by conventional SCS ( ). Importantly, serotonergic cells and OFF cells in the RVM were activated by SCS in only SCS responding rats with neuropathic pain (responders) and not in SCS nonresponding rats ( ). These responders also show an increase in 5-hydroxytyptamine (5-HT) levels in the lumbar DH following SCS ( ). Before this study, a release of 5-HT by SCS was demonstrated in cats ( ). The role of LC in SCS analgesia is less clear. LC contains many noradrenergic cell bodies and SCS increases LC neuronal activity, but it does not increase the noradrenaline content of spinal cord. Furthermore, blocking spinal noradrenergic receptors and inhibiting ipsilateral LC neurons does not reduce SCS-induced pain inhibition ( ). It remains possible that LC neurons send cortical projections and contribute to other therapeutic actions of SCS. Conventional SCS may inhibit cortical pain processing through ascending pathways relayed by thalamocortical systems ( ). These supraspinal mechanisms may work in concert with spinal mechanisms to reduce the descriptive and affective components of pain and improve quality of life ( ).
Burst SCS may act through supraspinal mechanisms by modulating cortical areas involved in pain modulation ( ). It was suggested that both burst and tonic SCS modulate the descending pain inhibitory system, as well as a self-referential, contextual, aversive memory system ( ). Findings from source-localized electroencephalographic subtraction and conjunction analysis in patients suggest that burst SCS could activate cortical areas, such as the dorsal anterior cingulate cortex (ACC) and the right dorsolateral prefrontal cortex, that are involved in the modulation of pain perception ( ). While some cortical areas such as the pregenual ACC can be activated by both burst and tonic SCS, only burst SCS reduces the connectivity between the dorsal ACC and the para-hippocampal cortices. Thus, burst SCS may normalize the pain–supporting/suppressing balance in contrast to tonic SCS by a greater effect on the dorsal ACC. It was further hypothesized that burst SCS may modulate the medial pain pathway directly by actions onto lamina I projection neurons targeting the dorsomedial nucleus of the thalamus. Nevertheless, research on eventual participation of supraspinal circuitry in burst SCS is ongoing, and the stimulation paradigm itself might be subject to change in the near future. It remains unclear whether pain inhibition by subliminal HF-SCS may involve any supraspinal mechanism.
Stimulation Parameters
Charge delivery may determine the therapeutic effect of SCS paradigms ( ). A greater charge delivery rate has been postulated to contribute to the greater inhibitory effect of burst SCS compared with conventional, tonic SCS ( ). Increasing evidence suggests that the mechanisms of pain inhibition by paresthesia-free HF-SCS and burst SCS differ from that of conventional SCS ( ).
Central neurons are sensitive to changes in surrounding electric fields ( ). Small electric fields can silently modulate neuronal excitability in a subthreshold manner and change neuronal encoding. Thus, stimulation that generates a weak electrical field around neural tissue may exert a therapeutic effect, even without sensory perception (e.g., paresthesia). This “weak, electrical, field modulation” may be a potential, segmental mechanism of pain relief for HF-SCS and burst SCS ( Fig. 15.1B and C ). Recent computer modeling provides a “differential blocking” hypothesis for HF-SCS ( ). Yet, this hypothesis is questionable against the clinical observations that neither sensory disturbance nor numbness seems to occur during 10-kHz HF-SCS (also see details in HF-SCS section). This makes a mechanism including selective blocking of large-diameter Aβ-fibers less likely. More biological studies are certainly needed to further elucidate these hypotheses.
Neurochemical Mechanisms
GABA
SCS increases extracellular GABA content in the spinal cord and is associated with decreases in glutamate and aspartate that exceed the duration of stimulation ( ). Inhibition of pain and decreased WDR neuronal hyperexcitability are closely associated with the time course of elevated GABA levels and decreased levels of glutamate and aspartate after SCS. The GABA B receptors may play a more important role than the GABA A receptors in mediating these inhibitory effects ( ). Furthermore, the intrathecal administration of a low dose of baclofen transforms SCS nonresponder rats into responders to SCS and enhances SCS-induced pain relief in human subjects ( ). However, effects of burst SCS do not rely on activation of GABA B receptors ( ) because GABA levels do not change after burst SCS and pain inhibition is not blocked by CGP35348, a GABA B receptor antagonist ( ). In SG neurons, blocking fast inhibitory neurotransmission mediated by GABA A and glycine receptors does not preclude the inhibition of C-fiber eEPSCs by 50-Hz Aβ-fiber stimulation ( ). The neurochemical mechanisms by which SCS inhibits excitatory and inhibitory interneurons are still incompletely known. It will be important to develop cell type-selective modulation and rationally choose adjuvant pharmaceutical agents to enhance SCS analgesia (e.g., agents to prevent the suppression of inhibitory neurons by SCS).
Serotonergic, Cholinergic, and Adrenergic Mechanisms
SCS induces release of serotonin into the spinal cord ( ), which decreases neuronal excitability and pain transmission by activating 5-HT 2A , 5-HT 3 , and 5-HT 4 receptors ( ). Serotonin may also increase the spinal expression and synthesis of dynorphin, enkephalin, and GABA ( ), which prolongs pain inhibition by SCS. SCS also induces the release of acetylcholine, adenosine, and noradrenaline ( ). Spinal acetylcholine content is elevated by SCS only in responder rats and not in nonresponder rats ( ). Acetylcholine and noradrenaline may excite spinal, GABAergic interneurons by binding to muscarinic M4 receptors and α 1 -adrenoceptors, respectively ( ). Intrathecally administered, subeffective doses of clonidine or a muscarinic receptor agonist were shown to transform SCS nonresponders into SCS responders ( ).
A recent study suggests that activation of spinal cannabinoid CB1 receptors may contribute to synaptic depression from high-threshold afferent inputs in SG neurons after electrical stimulation of Aβ-fibers in the DR. It may also be involved in conventional SCS-induced inhibition of spinal nociceptive transmission after nerve injury ( ).
Collectively, SCS may initiate feedforward activation of various spinal neurochemical mechanisms, some of which (e.g., GABA) may be compromised by nerve injury ( ). It remains to be determined if different neurochemical mechanisms exhibit synergistic functional interactions. Better understanding of the neurochemical basis for SCS analgesia will provide rationales for developing new treatment strategies that include the addition of adjuvant drugs to potentiate SCS analgesia ( ).
Other Mechanisms
In addition to the mechanisms just mentioned, SCS may induce transcriptional and posttranslational changes in neurons. Examples include activation of extracellular signal–regulated kinase (ERK) and AKT pathways ( ) and expression of the immediate early gene c- fos ( ). These changes may alter gene expression and exert long-lasting impact on cellular function, but the physiologic implications of SCS analgesia remain to be determined. High throughput approaches such as full-genome microarray analyses begin to reveal SCS-induced changes in a large number of genes in the spinal cord and DRG and uncover the highly significant interconnected genes and pathways that may be responsive to pain inhibition by SCS ( ).
High-Frequency Spinal Cord Stimulation
Background
Fundamental to the clinical success of paresthesia-based SCS is the concept that paresthesia (i.e., abnormal sensations caused by Aβ-fiber activation that includes what is often perceived by patients as tingling, buzzing, etc.) must be experienced as overlapping the patient’s painful areas to provide pain relief (also see introduction ) ( ). Traditionally, SCS has been administered at frequencies intending to provide a comfortable sensation for the patient, approximately 40–100 Hz ( ). Frequencies up to 1500 Hz have sometimes been explored in certain conditions. Waltz used frequencies ranging from 100 to 1500 Hz in the early 1980s when applying SCS for torticollis and spasticity, stating that therapeutic success was dependent on a unique and often narrow range of frequencies for any given patient ( ). Bennett et al. suggested that frequencies ranging from 250 to 1500 Hz could be successful in recovering pain relief in approximately 16% of CRPS subjects who retained paresthesia sensation in concordance with pain but had lost relief when using traditional lower frequencies ( ). Despite these clinical reports of the effect of higher frequencies, many preclinical and clinical SCS studies used frequencies of only 40–100 Hz. Instead, they focused on the effects of stimulation parameters that had been observed to alter paresthesia location and intensity, such as amplitude, pulse width, and contact location ( ). Reflecting this focus on stimulation parameters other than frequency, clinically available SCS devices, until 2010, had a frequency upper limit of 1200–1400 Hz ( ). For peripheral vascular disease, SCS has been used to increase peripheral blood flow. Gao et al. demonstrated preclinically in that increasing the frequency of SCS up to 500 Hz applied to the rat DCs enhanced peripheral blood flow. It was also demonstrated that these effects were probably due to antidromic presynaptic activation of small fibers containing the vanilloid receptor-1 (VR-1), thereby inducing a peripheral release of CGRP ( ).
The first publication of high-frequency (i.e., >1.5 kHz) SCS for pain treatment emerged in 2013, and 10-kHz SCS was shown to achieve pain relief without the inducement or need of paresthesia. Furthermore, 21 of 24 subjects who had trialed both low- and high-frequency SCS preferred 10-kHz SCS ( ). Results from a subsequent long-term 2-year follow-up prospective multicenter study indicated that 10-kHz paresthesia-free SCS resulted in robust responder rates (>50% pain relief) of 60% for back pain and 71% for leg pain, respectively, in patients with predominant back pain, a traditionally challenging patient population for conventional SCS ( ). In the first prospective randomized head-to-head study of SCS Kapural et al. confirmed these results, reporting that 10-kHz paresthesia-free SCS was statistically and clinically superior to conventional SCS at both 12- and 24-month follow-up, with responder rates for back and leg pain of 77% and 73% at 2 years, respectively ( ).
Mechanisms of Action of High-Frequency Spinal Cord Stimulation
Computational Modeling
In the spinal cord, computational models have explored the response of DC fibers to kilohertz stimulation frequencies. One study explored whether “depolarization blockade” of DC and/or DR fibers occurs with 1-kHz epidural SCS. These authors concluded that to achieve any blockade of the largest fibers, the stimulation amplitude must be approximately twice the activation threshold, similar to observations in peripheral nerve, and that the clinically reported amplitudes for 10-kHz paresthesia-free SCS were likely below the block threshold ( ). A different computational modeling investigation of kilohertz stimulation in SCS posed that DC blockade may be fiber selective ( ). Under the assumption that the tissue intervening between the SCS contact surface and the DC axons alters the shape of the stimulating pulse from symmetric biphasic to quasi-monophasic, Arle et al. calculated in a computational model of SCS that large-fiber blockade could be achieved at much lower amplitudes while still activating medium and smaller-diameter myelinated DC fibers. In these authors’ view, this might explain the lack of paresthesia, because the larger-diameter DC fibers would primarily communicate touch and pressure sensations from the periphery to higher centers (i.e., yielding perceived paresthesia), while the smaller-diameter fibers would be primarily proprioceptive and generate no sensation. Given that there are far more medium-sied and small fibers in the DC than large fibers, these proprioceptive fibers might then provide a more profound spinal gating of pain than activation of the relatively fewer large-diameter DC fibers ( ). However, there have been no clinically reported observations of altered sensorimotor function with 10-kHz paresthesia-free SCS, so significant blockade of sensory fibers and/or massive activation of proprioceptive fibers appears unlikely. Thus, this hypothesis remains to be validated in both preclinical and clinical settings.
Animal Studies
Preclinical investigations of kilohertz stimulation frequency to date have predominantly focused on the response of peripheral nerves, primarily exploring the phenomenon of “depolarization blockade.” For example, 20-kHz sinusoidal currents block activity in peripheral nerves ( ) and biphasic rectangular pulses induce block on motor nerve conduction ex vivo when high amplitudes are used at frequencies of 4 kHz and above ( ). Kilgore and Bhadra investigated neural blockade as produced by voltage-controlled and current-controlled stimulation by pulsatile and sinusoidal waveforms (among others) and monopolar versus bipolar electrode configurations and produced complete sciatic nerve conduction block over the frequency range of 2–20 kHz in adult bullfrogs ( ). Later, in studying motor fiber blockade in the rodent by using high-frequency sinusoidal alternating current, Bhadra and Kilgore demonstrated that stimulation between 10 and 30 kHz, at intensities between 2 and 10 V, produced complete repeatable and reversible motor block that showed a linear relationship to frequencies used ( ). Joseph and Butera also observed both A- and C-fiber blockade at kilohertz frequencies up to 50 kHz but stated that unmyelinated C-fiber blockade had a nonmonotonic relationship to frequency ( ). Cuellar et al. explored the effects on DH neurons on stimulation frequencies ranging from 2 to 100 kHz applied to DRs and DREZ and appeared to also have observed depolarization block of primary afferent neurons. They saw that responses to innocuous as well as nociceptive inputs were reduced at kilohertz frequencies with increasing stimulation amplitude ( ). Notable in the studies of kilohertz frequency depolarization blockade is the relatively high amplitude of stimulation required to achieve the block. Both computational modeling and preclinical studies in peripheral nerves have shown that the block threshold (i.e., the voltage or current amplitude of the stimulation) is approximately 2.5–5 times the activation threshold for the neuron ( ). Indeed, the axon to be blocked must generate at least one AP for the gating dynamics of the membrane ion channels to enter the state for a possible depolarization block ( ). It is believed that activation of K + channels or inactivation of Na + channels is responsible for the blocking effects of kilohertz-frequency nerve stimulation ( ). Clinically, attempts at peripheral nerve blockade have indicated that sensation tends to precede or accompany an assumed blockade of activity ( ).
Recent preclinical work looking into mechanisms of HF-SCS has yielded variable results. In preparations where the DC was targeted for stimulation, variations in frequency demonstrated behavioral changes only when relatively large amplitudes of stimulation were used. Shechter et al., using spinal nerve ligation rodent pain models, observed that only stimulation intensities of 40% or greater of motor threshold (MT) yielded significant increases in paw withdrawal threshold (PWT) for 50 Hz, 1 kHz, and 10 kHz when compared with sham stimulation ( ). In these series of experiments, 40% of MT was considered to be just below sensory threshold; the highest amplitude, 80% of MT, yielded even larger effects but may be considered too strong a stimulation intensity for clinical use. These behavioral effects were shown to be more pronounced with repeated 30-min applications of SCS over 3 days and by use of kilohertz frequency (compared with 50 Hz). Additionally, at very strong intensity, 50 Hz reduced the C-fiber component of WDR neuronal wind-up response compared with 1 kHz. , in both spared nerve injury and inflammatory pain model rats, explored behavioral effects of 50 Hz, 500 Hz, 1 kHz, and 10 kHz and made efforts to program the stimulation to intensities believed reflective of clinical practice: 80% of MT for 50 Hz and 40–50% of MT (“subparesthetic intensity,” determined by behavioral observations and corroborated by companion, electrophysiologic measurements in nucleus gracilis) for the higher frequencies ( ). Stimulating for 2 h, they saw large early changes for 50 Hz compared with other frequencies, but by the end of the stimulation period, all frequencies yielded similarly, significant improvements in mechanical (but not thermal) PWT in the affected limb compared with the contralateral limb. In a follow-up study, Song et al. explored the importance of pulse shape and pulse width for 50-Hz and 1-kHz frequencies ( ). They found similarly that 50 Hz, at PW = 200 μsec at 80% MT provided reductions in PWT earlier in the stimulation protocol, but after 60 min of stimulation, monophasic and biphasic 1-kHz stimulation with a negative phase of 24 μs (but not 12 μsec) at 40–50% MT showed similar reductions in PWT. The lack of a distinct response to kilohertz frequency in these DC-focused preclinical studies suggests that different models and neural targets may be necessary to uncover HF-SCS mechanisms, as discussed in “Future Directions” later.
Summary
The lack of paresthesia, yet durable efficacy, suggests that 10-kHz SCS may either amplify existing modes of action for low-frequency SCS or perhaps operate through different mechanisms, either as adjunct or independently. Indeed, given the variable results of preclinical investigations of DC-focused, kilohertz SCS discussed earlier, newer concepts of neural targeting might provide better hypothetical frameworks for exploration of high-frequency mechanisms. One such proposal is that DC-focused SCS may not apply to clinical 10-kHz paresthesia-free SCS. Compared with paresthesia-based, low-frequency SCS, there are two key differences in the clinical techniques for 10-kHz paresthesia-free SCS: (1) leads are positioned anatomically, spanning T8–T11, without intraoperative paresthesia mapping and (2) programming is not based on paresthesia concordance with pain but rather on direct assessment of pain relief from the 10-kHz stimulation ( ). In subjects successfully using 10-kHz paresthesia-free SCS, pain relief was uncorrelated to paresthesia overlap from low-frequency suprasensory SCS at stimulation sites optimized for 10 kHz ( ). Additionally, pain relief outcomes with 10-kHz paresthesia-free SCS were not statistically different between subjects with leads positioned on the same side as predominant pain and those subjects with leads positioned opposite to the side of their predominant pain ( ). Both of these results suggest that 10-kHz paresthesia-free SCS is actually “paresthesia independent” and the DCs may therefore not be the primary target of the stimulation field ( ).
Use of the Electrically Evoked Compound AP to Study Types of Fibers Activated by SCS
Background
The electrically evoked compound action potential (ECAP) is a direct measure of the response of neurons to a stimulus. It is the sum of all single-fiber APs in a population of neurons. In response to therapeutic stimulation, the axons of the DC produce a triphasic ECAP, which consists of the P1, N1, and P2 peaks ( ). The ECAP propagates orthodromically and antidromically, and the timing of the peaks allows the calculation of conduction velocity ( ). The amplitude of the ECAP and its decay can be used to estimate the distribution of conduction velocities, and hence fiber sizes, in the responding fibers ( ). Paired-pulse and other more-complex pulse experiments reveal the dynamic response of the axons to a variety of stimulation parameters. The opening and closing of ion channels and the passage of ions across the membranes of the axon, which occurs during depolarization, produce the electric field that is recorded during ECAP measurement, and, as a result, the measurements provides information about the ion channels of the responding axons.
Understanding the neurophysiologic response of the DC to stimulation under a wide range of stimulation parameters is a necessary and first step toward defining an MOA for SCS. The pain amelioration produced by SCS depends on the generation of electrical neural activity and subsequent neurochemical responses. Quantifying the response to the stimulation is a measure of the activity and an indirect measure of activity-generated neurochemical response. With careful design, electrophysiologic experimentation can reveal the details of spinal cord neuron responses under a variety of stimulating conditions. Here, we shall look at findings elucidated by measuring ECAPs within the context of the prevailing literature.
ECAP Measurement
Modern SCS devices use multichannel linear electrode arrays where the contacts on the array are separated from each other by a fixed distance (a typical lead has eight contacts with intercontact spacing of 7 mm). ECAPs can be measured on the nonstimulating electrodes ( Fig. 15.2 ).
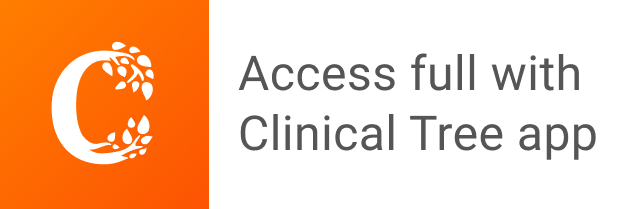