Stroke occurs when blood flow to the brain is disrupted and the areas of brain deprived of oxygen die. This can be caused by obstruction or hemorrhage of blood vessels.
Loss of oxygenation causes death of neurons through complex processes, which include excitotoxicity, mediated by excessive release of glutamate from damaged neurons, and subsequent increases in intracellular calcium levels and overactivation of calcium-dependent enzymes.
Loss of oxygenation also causes neuronal death through the formation of free radicals and through genetically programmed cell death, called apoptosis.
Despite increased understanding of the biochemical processes underlying neuronal death, the best therapy for stroke remains rapidly restoring the brain’s blood supply and preventing the formation of clots and emboli.
This includes the use of antiplatelet agents such as aspirin and clopidogrel, and oral anticoagulants such as warfarin and dabigatran, as well as thrombolytic agents such as tissue plasminogen activator (tPA), which target different steps of coagulation cascades.
Migraine headaches are believed to result from waves of inhibitory neuronal activity called “cortical spreading depression” that stimulate trigeminal nerve endings innervating the brain’s vasculature. This causes release of proinflammatory substances, such as calcitonin gene–related peptide (CGRP), into and around the vessels, resulting in vasodilation and pain.
Treatment regimes for migraine headaches typically employ both prophylactic and abortive strategies.
The mainstay in abortive treatment is the triptan drugs, such as sumatriptan, which are agonists at serotonin 5HT1B and 5HT1D receptors.
Vertebrate neurons are exquisitely specialized for the functions they perform. As explained in previous chapters, a single neuron may receive information from and relay information to thousands of other neurons; consequently, the nervous system is capable of remarkably complex functions. Moreover, the brisk flux of ions across neural membranes permits extremely rapid interneuronal signaling. However, this specialization comes at a cost. A tremendous amount of energy is required to maintain ionic gradients across the membranes of the approximately 100 billion neurons that comprise the human brain. Although the brain represents only 2% of the body’s total mass, it uses approximately 20% of the body’s oxygen supply, and blood flow to the brain accounts for about 15% of total cardiac output. Ischemia, or insufficient blood supply, results in oxygen and glucose deprivation and in the buildup of potentially toxic metabolites such as lactic acid and CO2. Interruption of blood flow to the brain can lead to complete loss of consciousness within 10 seconds, the approximate amount of time required to consume the oxygen contained in the brain.
Stroke occurs on disruption of blood flow to brain tissue caused by obstruction of blood flow or bleeding in the brain (hemorrhage). The exquisite vulnerability of neurons to energy deprivation caused by stroke results in vast medical, economic, and personal costs. In the United States alone, roughly 795,000 strokes occur each year. This equates to an average of one stroke every 40 seconds in the American population. Approximately 150,000 of these strokes are fatal, which equates to one death every 4 minutes, making stroke the fourth leading cause of death in the United States. Survivors of stroke often are beset by serious long-term disabilities, including paralysis and disruption of higher cognitive functions such as speech. Individuals with such disabilities may be unable to resume work and other daily activities, and often require extensive long-term care by healthcare professionals or friends and family.
The term stroke, now less commonly referred to as a cerebrovascular accident (CVA), broadly refers to neurologic symptoms and signs that result when blood flow to brain tissue is interrupted. The two primary types of stroke, as noted above, are occlusive and hemorrhagic. An occlusive stroke is caused by the blockage of a blood vessel and accounts for 87% of all strokes in the United States 20–1. Vascular occlusion, which generally restricts blood flow to a discrete area of the brain, results in neurologic deficits and in a loss of functions controlled by the affected region. Occlusive strokes typically are caused by embolic, atherosclerotic, or thrombotic occlusion of cerebral vessels 20–2.
20–1
Critical stenosis of the internal carotid artery (ICA) at the bifurcation of the common carotid artery (CCA) into the ICA and external carotid artery (ECA). Shown is critical (>90%) stenosis of the ICA identified on digital subtraction angiography in a patient with a recent left hemispheric stroke. (Used with permission from Robert Bucelli, Washington University School of Medicine.)

20–2
Embolic infarctions. Diffusion-weighted MRI revealing embolic infarcts (white) within the left middle cerebral artery (MCA) territory. Such emboli may have arisen from blood clots in the heart (cardioembolic) or from clots or atheromatous plaque from an artery such as the carotid (artery-to-artery emboli).

A hemorrhagic stroke is caused by bleeding from a vessel and accounts for 10% of all strokes in the United States. Intracranial bleeding can occur in the intraparenchymal, epidural, subdural, or subarachnoid spaces. Intraparenchymal hemorrhage may be caused by acute elevations in blood pressure or by a variety of disorders that weaken blood vessels. Chronic hypertension is the most common predisposing factor, but coagulation disorders, brain tumors that promote the development of fragile blood vessels, amyloid deposition in blood vessels (ie, amyloid angiopathy; Chapter 18), and the use of cocaine or amphetamines—both of which cause rapid elevation of blood pressure—are among the risk factors for intraparenchymal hemorrhages. Intraparenchymal hemorrhaging can lead to the formation of blood clots (hematomas) in the cerebrum, cerebellum, or brainstem, which in turn may limit the blood supply to nearby brain regions and exacerbate the injurious effects of a stroke. Hemorrhagic stroke can also occur secondary to an initially ischemic stroke.
Epidural, subdural, and subarachnoid bleeding often results from head trauma or the rupture of an aneurysm. Subarachnoid hemorrhages account for the remaining 3% of strokes in the United States. In addition to the damage caused by the loss of blood supply to affected areas of the brain, hemorrhages can cause damage by increasing intracranial pressure that further compromises neuronal health. Moreover, through mechanisms that are not completely understood, subarachnoid hemorrhage can cause reactive vasospasm of cerebral surface vessels several days to weeks after the hemorrhage, which in turn can lead to a further reduction in blood supply and additional cerebral infarcts.
When neurons are deprived of the nourishment they require, they quickly become unable to maintain their resting membrane potentials and, as they depolarize, they fire action potentials. Their firing triggers the release of neurotransmitters, in particular, glutamate, which in turn promotes depolarization of neighboring neurons. Such activity sets the stage for a destructive cycle of neuronal activation, neurotransmitter release, and further activation. Prolonged periods of neuronal activation can lead to the disruption of ionic gradients, massive Ca2+ influx, cellular swelling, activation of cellular proteases and lipases, mitochondrial damage, generation of free radicals, and eventually widespread neuronal death. Ischemia of only a few minutes’ duration can result in permanent brain damage. The basic biochemical mechanisms responsible for these processes of neuronal injury and death, broadly termed either necrosis or apoptosis (programmed cell death), are described in detail in Chapter 18.
Despite our growing knowledge of the mechanisms that underlie ischemic neuronal death, our ability to treat stroke remains limited. Among the treatment strategies currently available, the best are geared toward prevention through the maintenance of cardiovascular health, restoration of blood supply, and the slowing of metabolism with hypothermia. Although none of these therapies has capitalized on the sophisticated studies of the biochemical events underlying neuronal cell death, efforts to prevent stroke nevertheless have been successful. The incidence of stroke has been reduced markedly by primary preventive measures aimed at controlling hypertension, hypercholesterolemia, diabetes, and tobacco use. HMG-CoA reductase inhibitors, known as the statin class of cholesterol-lowering agents, appear to confer additional benefit in stroke prevention beyond cholesterol control, as treatment with statins in individuals with normal cholesterol levels significantly reduces the incidence of future stroke. Prophylactic use of drugs that inhibit platelet function, such as aspirin, clopidogrel, or dipyridamole, has proven to be effective in reducing the risk of occlusive stroke. While these drugs can result in a slight increase in the risk of hemorrhagic stroke, this small risk is outweighed markedly by the benefit conferred for ischemic stroke prevention.
One reason that stroke prevention is so important is that current stroke therapies are pitted against an unforgiving opponent: time. “Time is brain” is a fundamental concept to the treatment of acute ischemic stroke. As previously emphasized, serious neuronal damage can occur within minutes of an ischemic event. Barring round-the-clock observation of all individuals at risk for stroke, the effectiveness of treatment in humans is unlikely to approach that of laboratory animals because researchers in the laboratory have the luxury of administering therapy during or immediately after an ischemic insult. This underscores the need for protective therapy in high-risk populations.
Many of the approaches to treatment discussed in the sections that follow involve actions that occur primarily in the peri-infarct area, the “penumbra,” and serve to salvage at-risk neurons that would otherwise be destined to die within hours or days after a stroke. The peri-infarct area constitutes compromised but potentially salvageable tissue between the severely ischemic core and adequately perfused brain tissue. Although potentially salvageable, the peri-infarct area is quite vulnerable because it is subject to high levels of excitotoxic neurotransmitters and free radicals, waves of cellular depolarization, and inflammatory processes (Chapter 18).
After an occlusive stroke, blood supply can be restored with thrombolytic agents, which dissolve the clots that impede the normal flow of blood. Thrombolytic agents have been found to improve the outcome of this type of stroke in clinical trials. However, strict protocols are utilized in determining patient eligible for this form of therapy in order to minimize the risk of hemorrhage. Consequently, the presence of hemorrhagic stroke must be ruled out by use of computerized tomography (CT), and other risk factors such as malignant hypertension, recent surgery, or prior cerebral hemorrhage must be excluded before thrombolytic agents are used. Even occlusive strokes are accompanied by a small but real risk of hemorrhagic transformation.
Thrombolytics such as tissue plasminogen activator (tPA; eg, alteplase, reteplase), urokinase, streptokinase, prourokinase, and desmoteplase are proteins that promote the conversion of the proenzyme plasminogen into plasmin, an enzyme that degrades fibrin, a key structural protein in most blood clots 20–3; 20–1. Currently, tPA is the only thrombolytic substance approved for intravenous use in acute ischemic stroke in the United States. Clinical trials demonstrate that intravenously delivered tPA reduces the disability of patients with acute ischemic stroke who were treated within the first 4.5 hours of the onset of symptoms.
20–3
Mechanisms of action of anticoagulants and thrombolytics. A. Platelets are activated by molecules exposed during tissue injury. Aspirin inhibits cyclooxygenase, which catalyzes the formation of thromboxane A2, a key intermediary in the clotting process. Clopidogrel and related drugs antagonize the activation by ADP of platelet P2Y12 receptors, which promote platelet aggregation. Dipyridamole inhibits clot formation through mechanisms as yet unknown. B. During the clotting cascade, a chain of precursor proteins (mostly serine proteases) activate one another, a process that results in amplification of the signal. Heparin activates the endogenous protein, antithrombin III, which then inhibits several of the activated clotting proteins, in particular, factor Xa and thrombin (factor IIa). Warfarin and related agents deplete vitamin K–dependent clotting factors: factors Xa, IXa, and VIIa, and thrombin. Apixaban and rivaroxaban inhibit factor Xa, while dabigatran inhibits thrombin. C. Thrombolytics such as tissue plasminogen activator (tPA) and streptokinase catalyze the conversion of the inactive precursor plasminogen to the active enzyme plasmin, which catalyzes the breakdown of fibrin polymers. Fibrin is a key component of the clot; it is produced from its precursor fibrinogen through catalysis by thrombin, a major product of the clotting cascade.

20–1 Role of Nitric Oxide in Stroke
Nitric oxide (NO) functions as an intracellular and intercellular messenger in the brain (Chapter 8). It is synthesized by the Ca2+-activated enzyme, nitric oxide synthase (NOS). The importance of NOS activation in neuronal injury has been tested through the use of specific NOS inhibitors, such as L-nitroarginine. Initial studies produced inconsistent results: NOS inhibitors displayed neuroprotective effects in some experiments, especially those conducted in cell culture, and produced either no effect or a detrimental effect in others. These conflicting results most likely were attributable to the multiple roles and sources of NO in the brain.
Three different isoforms of NOS exist, each of which is the product of a distinct gene. Neuronal NOS (nNOS) is expressed exclusively in neurons, endothelial NOS (eNOS) originally was identified in endothelial cells, and inducible NOS (iNOS) originally was identified in certain immune system cells. Some neurons express eNOS and iNOS in addition to nNOS. NO produced by eNOS acts as an endothelial relaxing factor: it promotes the relaxation of the smooth muscle surrounding arterioles and leads to vasodilation and increased blood flow.
Intravenous treatment with the NOS substrate, L-arginine, promotes functional recovery in an experimental stroke model (see 20–6). A. Occlusion of the middle cerebral artery (MCA; onset indicated by red lightening) results in a profound reduction in regional cerebral blood flow (measured by laser Doppler flowmetry; lower graph) and functional activity of the brain (measured by electrocorticogram; upper graph). Days later, a large cerebral infarct evolves in the ischemic middle cerebral artery territory (indicated in red on the coronal brain section). B. In comparison, intravenous infusion of the eNOS substrate L-arginine after MCA occlusion in another rat augments cerebral blood flow, improves functional activity, and reduces the area of infarct compared with control treatment. These findings suggest that augmenting NO bioavailability can promote functional recovery in the ischemic brain. (Adapted with permission from Dalkara T, Morikawa E, Panahian N, et al. Blood flow-dependent functional recovery in a rat model of focal cerebral ischemia. Am J Physiol. 1994;267:H678–H683.)

Gene knockout technology has helped to elucidate the roles of these NOS isoforms in neuronal injury. Compared with their wild-type counterparts, mice deficient in nNOS typically have smaller infarct volume (ie, amount of necrotic tissue) after an experimentally induced ischemic stroke. This finding suggests that nNOS activity may be detrimental to neuronal survival during ischemia. In contrast, mice deficient in eNOS tend to have greater than normal infarct volumes after experimentally induced stroke, which indicates that eNOS has neuroprotective activity. Most likely, eNOS exerts its beneficial effect by promoting the reperfusion of the ischemic area. This is demonstrated in the figure. Interestingly, iNOS knockout mice, like nNOS knockout mice, display diminished infarct volume after ischemic stroke; it is speculated that a decreased inflammatory response may reduce infarct size in these mice.
The multiple effects of NO on ischemic injury provide an excellent lesson in the complexity of the brain’s response to ischemia. Other events that take place during ischemia, such as Ca2+ entry into cells, also may have multiple and varied effects, and these actions must be carefully examined if effective therapies are to be devised. If, for example, NOS inhibition can be developed as a clinical treatment for ischemic neuronal injury, such inhibition most likely will have to be carefully targeted to nNOS or perhaps iNOS.
Prior to large initiatives to expedite the treatment of acute ischemic stroke, similar to efforts for acute myocardial infarction, by the time an individual was aware of the occurrence of a stroke, traveled to a hospital, and was diagnosed, hours had elapsed. In many centers around the world, the average onset to treatment time has dropped to under 60 minutes. However, despite these marked improvements in select centers, and the fact that administration of intravenous tPA within 4.5 hours of symptom onset is now standard of care in the United States, only 3% to 5% of stroke patients actually receive tPA because of the difficulty of administering it within this time frame.
Even when intravenous tPA is successfully administered in time, the affected vessel does not always open or open completely. At academic centers, intravenous therapy is sometimes followed by interventional procedures, where a catheter is guided from a peripheral artery (usually the femoral artery in the groin) to the affected cerebral artery. The clot is then treated with a combination of mechanical clot disruption or direct instillation of a thrombolytic agent into the clot itself. Because a smaller dose of the thrombolytic agent is used when given at the site of the clot itself, as compared with systemic (intravenous) administration, intra-arterial therapy is in theory safer and can be performed up to 8 hours after symptom onset. However, three large trials published in 2013 argued against widespread use of this approach due to negative results. Thus, current recommendations are for intra-arterial therapy to be used on a selective, case-by-case basis. In cases of basilar artery thrombosis, when the brainstem is at risk and the matter of opening the artery is literally life and death, intra-arterial therapy administered up to 48 hours after symptom onset may improve outcomes. Prourokinase, urokinase, and desmoteplase are additional thrombolytics that are used less commonly in intra-arterial therapy.
Heparin is a heterogeneous mixture of sulfated mucopolysaccharides. It is found in mast cells and in the extracellular matrix of most tissues. It has a molecular mass of 750 to 1000 kDa and is composed of long polymers of glycosaminoglycan chains that are attached to a core protein 20–4. Because of its structure, heparin is not effective after oral administration and must be given parenterally. It inhibits clot formation by enhancing the activity of antithrombin III, a protein that forms equimolar complexes with the various proteases activated during the clot formation process (see 20–3). By binding directly to antithrombin III, heparin causes a conformational change in the protein that enhances its binding to the clotting factor proteases. Heparin has been shown to cause more harm than benefit as an acute treatment of stroke and carries a risk for increased hemorrhage.
As discussed in Chapters 4 and 11, aspirin inhibits cyclooxygenase, which in platelets catalyzes the conversion of arachidonic acid to thromboxane A2, among other products 20–4. Thromboxane A2 is a critical intermediate in the recruitment of platelets necessary for the clotting cascade. Aspirin administered to patients during hospital admission for stroke produces a small but significant net benefit in that it reduces mortality by 14% compared with placebo. Clopidogrel and related thienopyridine class agents (eg, prasugrel and ticlopidine) are other antiplatelet agents in current use. They act as adenosine diphosphate (ADP) antagonists, whereby they inhibit the binding of ADP to P2Y12 receptors (Chapter 8) on platelet membranes. They also irreversibly modify platelet P2Y12 receptors, and therefore their effects last for the lifespan of the platelets (approximately 7–10 days). The P2Y12 receptor is responsible for activation of the glycoprotein GPIIb/IIIa complex (also known as integrin αIIbβ3), the major receptor for fibrinogen.
Dipyridamole, used for similar purposes clinically, inhibits clot formation and causes vasodilation, although it is not known which of its many actions (eg, phosphodiesterase, adenosine reuptake, or adenosine deaminase inhibition) is responsible for the drug’s clinical effects. A combination drug of aspirin–dipyridamole has been shown to offer added benefit in stroke prevention, relative to either drug given alone. Recent evidence suggests that dual antiplatelet therapy with aspirin and clopidogrel may also have added benefit in select populations. However, combination therapy historically has been avoided due to the increased risk of hemorrhage reported in the MATCH clinical trial.
Patients at risk for cardioembolic strokes, such as those with atrial fibrillation or a mechanical heart valve, conditions that predispose patients to form intracardiac clots, often are treated with warfarin or one of the more recently developed oral anticoagulant alternatives (eg, dabigatran, rivaroxaban, and apixaban) 20–4. Warfarin is a synthetic derivative of a related compound in sweet clover, which was found in the early 20th century to promote bleeding. It acts as a functional vitamin K antagonist; it does not directly antagonize the function of vitamin K; rather, it depletes vitamin K by inhibiting its recycling. Vitamin K is a required cofactor for the enzymes that activate several clotting factors, including II, VII, IX, and X (see 20–3). Warfarin and related compounds are the most potent oral anticoagulants known; indeed, they are so potent that severe hemorrhage is a significant side effect of their use. (This effect, at high doses, is exploited in warfarin’s use as a rat poison.) Patients who take warfarin must have regular blood tests to ensure that their bleeding times are within safe boundaries and that dose adjustments are made accordingly. Unlike warfarin, the newer, oral anticoagulants do not require monitoring, allowing for standardized dosing; importantly, they appear equally efficacious. These drugs each target a specific factor in clotting cascades: dabigatran is a direct inhibitor of thrombin, while rivaroxaban and apixaban are inhibitors of factor Xa (see 20–3).
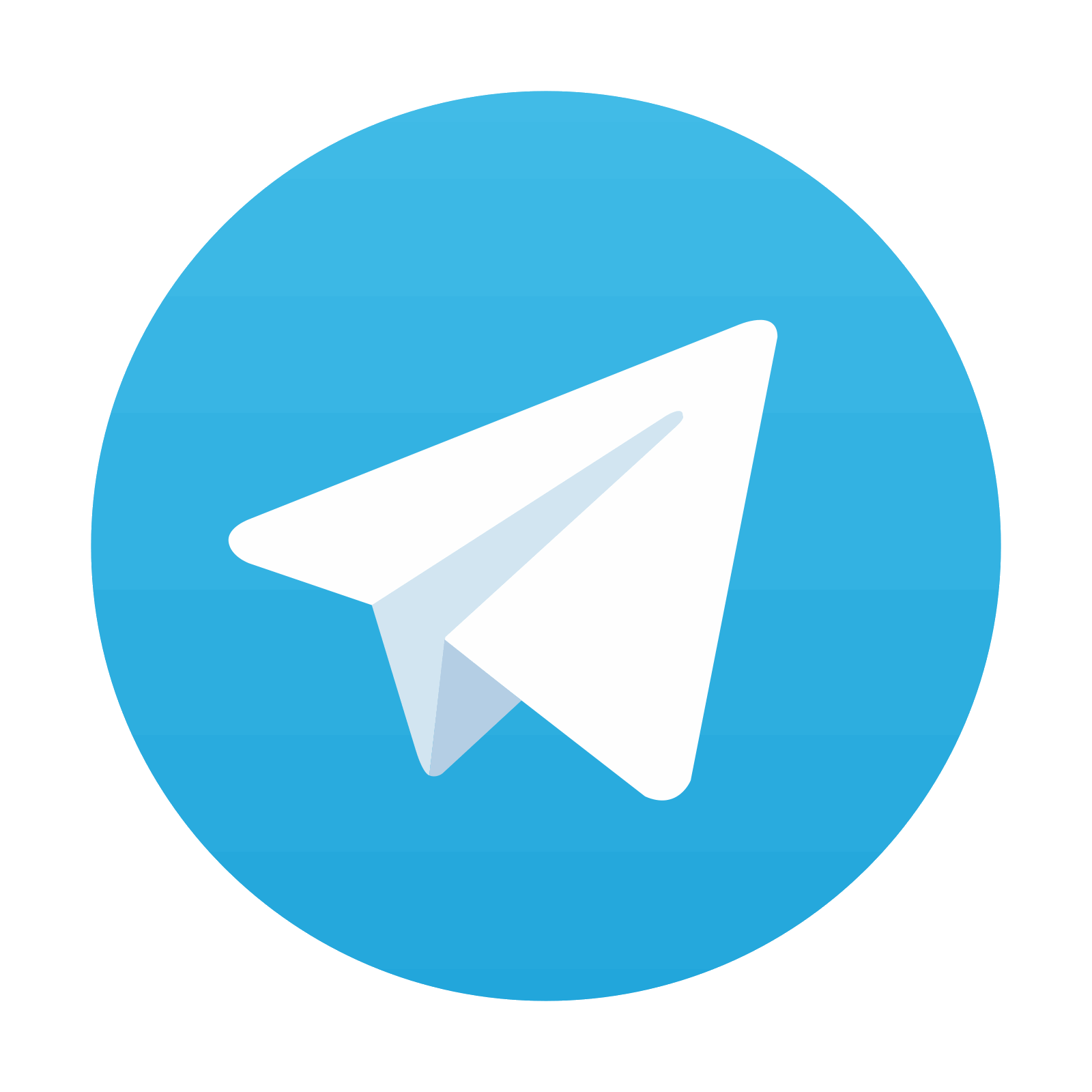
Stay updated, free articles. Join our Telegram channel
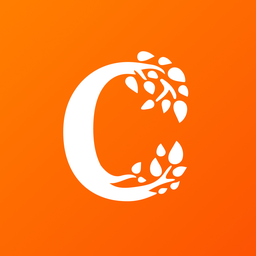
Full access? Get Clinical Tree
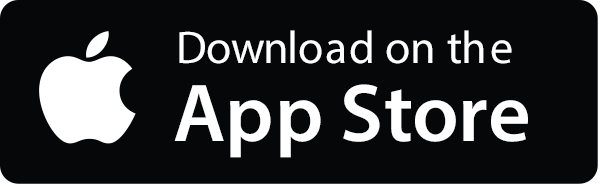
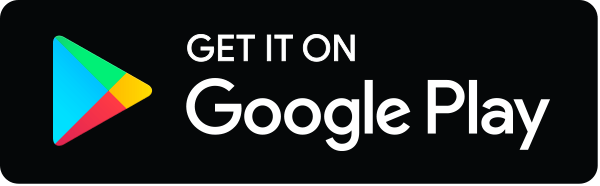