Synaptic transmission is a signal transduction process that begins with the action potential–dependent release of a neurotransmitter from a presynaptic terminal. The neurotransmitter then binds to and activates postsynaptic receptors that modify the electrical and biochemical properties of the postsynaptic cell.
The major classes of neurotransmitters are amino acid transmitters, such as glutamate and GABA; monoamines, including dopamine, norepinephrine, and serotonin; acetylcholine; peptides; diffusible gases, such as nitric oxide; lipid-derived molecules, such as endocannabinoids; and nucleosides and derivatives, such as adenosine and ATP.
Neurotransmitters are stored in small organelles called synaptic vesicles that fuse with the presynaptic terminal membrane and release their contents when an action potential invades the terminal and causes a rise in calcium due to activation of voltage-dependent calcium channels.
A single neurotransmitter typically activates several different subtypes of receptors.
Neurotransmitter receptors are classified as ligand-gated ion channels or G protein–coupled receptors.
After being released, most neurotransmitters are transported back into the presynaptic terminal or into glia by specialized proteins called plasma membrane transporters. A different family of transporters is responsible for pumping neurotransmitter into synaptic vesicles.
Neurotransmitter transporters are important targets of many antidepressant medications and psychostimulant drugs such as cocaine and amphetamines.
The proteins that are responsible for the fusion of synaptic vesicles with the presynaptic plasma membrane, a process known as exocytosis, have been identified and extensively characterized. Some of these proteins are the targets of bacterial toxins (eg, tetanus and botulinum toxin) and black widow spider venom (α-latrotoxin).
After exocytosis, synaptic vesicles are recycled and used again by repackaging them with neurotransmitter.
When we think, feel, or move, information passes rapidly between neurons across specialized gaps called synapses. When we learn and remember, synapses undergo significant activity-dependent alterations. Given the centrality of synaptic transmission to the function of the nervous system, it is not surprising that the large majority of drugs used to treat neuropsychiatric illnesses act on different protein components of specific synapses. This chapter explores the biochemical basis of synaptic transmission and explains how this process is regulated.
Neurons are morphologically specialized to receive, process, and send information 3–1. As discussed in Chapter 2, the key structure across which information is transferred, by use of chemical neurotransmitters, is the synapse. Synaptic transmission is a result of three types of processes that convert electrical information into a chemical signal and then back again: (1) electrical information in the axon of a presynaptic neuron is converted to a chemical signal in its nerve terminal, (2) this chemical signal is transmitted to another cell across a synapse, and (3) the chemical message received by the postsynaptic cell is converted into an electrical signal plus a range of chemical signals. It should be noted that a small percentage of synapses are gap junctions, morphologic connections between two neurons that permit the direct flow of electrical current from cell to cell (Chapter 2). These electrical junctions are morphologically distinct from chemical synapses. Because virtually all pharmacologic agents act at chemical synapses rather than at gap junctions, chemical synapses are the sole focus of this chapter.
3–1
At the interface between an axon and a postsynaptic cell, known as the synaptic cleft, an axon terminal, or synaptic bouton, innervates a dendritic spine. In the active zone of the presynaptic terminal, synaptic vesicles cluster against the plasma membrane. A reserve pool of synaptic vesicles lies nearby, and the postsynaptic density lies opposite the active zone within the dendritic spine.

Most types of communication between neurons must be precise and rapid. Fast and accurate information exchange is partly ensured by restricting the process within a synapse. The synapse was originally defined by three distinct elements: a presynaptic nerve terminal or bouton, its postsynaptic target, and the space between them known as the synaptic cleft. Over the past several decades, extensive investigation has revealed additional details, including presynaptic structures specialized to release chemical neurotransmitters from the nerve terminal, and postsynaptic structures involved in processing these signals 3–1 and 3–2. On the presynaptic side of the synapse, in the active zone of the nerve terminal, neurotransmitter is released from vesicles that cluster near the plasma membrane. On the other side of the synapse are postsynaptic specializations, the composition of which varies with the type of neurotransmitter released from the presynaptic terminal. The postsynaptic density, which is detected at excitatory synapses, is the best-characterized postsynaptic specialization. It is easily identified in electron micrographs 3–2 as a proteinaceous matrix of fine filaments just under the plasma membrane of postsynaptic dendritic spines. The proteins that comprise this structure, such as postsynaptic density protein 95 (PSD95), act as a scaffold to cluster and organize molecules involved in postsynaptic signaling. The synaptic cleft itself is bound by extracellular matrix proteins and cell adhesion molecules that may function to strengthen or weaken synaptic contacts.
3–2
Electron micrograph of an excitatory synapse. Two primary components are evident: a presynaptic terminal (right) and the postsynaptic target, with a dendritic spine to the left. A depolarization-dependent influx of Ca2+ into the terminal causes synaptic vesicles, visible as uniform spherical organelles, to fuse with the plasma membrane, thereby releasing neurotransmitter into the synaptic cleft. Released transmitter subsequently binds to postsynaptic receptors. The postsynaptic density, the site at which receptors are located, is visible as the dark, electron-dense region adjacent to the plasma membrane at the end of the dendritic spine. (Used with permission from K. Harris from Synapse Web: synapses.clm.utexas.edu.)

Neurotransmitters are often classified by their chemical makeup, although there is only a rough correlation between these classes and neurotransmitter function 3–1. The major chemical groupings are amino acid transmitters, such as glutamate and γ-aminobutyric acid (GABA); monoamines, including dopamine, norepinephrine, and serotonin; peptides; diffusible gases, such as nitric oxide; nucleosides and derivatives, such as adenosine and adenosine triphosphate (ATP); and lipid-derived signaling molecules, such as endogenous cannabinoids (endocannabinoids). Historically, it was believed that only one type of transmitter could be synthesized and released from a cell, but we now know that a single neuron may utilize multiple neurotransmitters. Examples include corelease of monoamines such as norepinephrine with peptides such as neuropeptide Y, but some neurons also release more than one small-molecule neurotransmitter, and some neurons release multiple neuropeptides (Chapter 7). The biosynthesis, metabolism, and functions of the different types of neurotransmitters, as well as the receptors that mediate their signaling, are discussed extensively in subsequent chapters. This chapter focuses predominantly on the mechanisms underlying neurotransmitter release.
With the exception of the diffusible gases and lipid-derived signaling molecules, most chemical transmitters are stored in secretory vesicles. Like other cellular organelles, these vesicles have a limiting membrane composed of a phospholipid bilayer and an aqueous lumen 3–1. The lumen is filled with thousands of transmitter molecules that sometimes reach near-molar concentrations. During synaptic transmission, secretory vesicles fuse briefly with the plasma membrane, allowing communication between the aqueous interior of the vesicle and the extracellular space. The most abundant form of secretory vesicle in the central nervous system (CNS) is the so-called small synaptic vesicle. These vesicles cluster in the active zone of the nerve terminal and normally release all or most of their contents rapidly when the nerve terminal depolarizes.
3–1 Membrane Bilayers and the Phosphatidylinositol System
Membrane bilayers serve to compartmentalize cells; however, they are fluid and flexible. As a result, membrane-defined vesicles can fuse with a plasma membrane and can form by budding from parent organelles. Furthermore, the membrane bilayers surrounding different organelles vary in terms of their phospholipid composition, and the head groups of the component lipids can be altered enzymatically. The 1, 4, and 5 positions of the inositol head groups may be linked to phosphate groups by reactions that are catalyzed by different lipid kinases (see figure). In addition, lipid phosphatases can remove phosphate from the inositol ring. A growing number of observations link phosphatidylinositol (PI) phosphorylation to membrane fusion events.
Because phospholipases can cleave chemical bonds in phospholipids, they play an important role in cell signaling and membrane trafficking. Two products of phospholipase C and D (PLC and PLD) are shown in the figure. The products of PLC, inositol trisphosphate (IP3) and diacylglycerol (DAG), are important second messengers and play a role in cell signaling as discussed in Chapter 4. In contrast, the product of PLD, phosphatidic acid (PA), appears to be involved in membrane trafficking. Studies have revealed that IP3 can activate PLD, thus indicating the complex functional interaction between these pathways.
The amount of transmitter in each vesicle is called a quantum. Because transmitter release proceeds through the fusion of individual vesicles with the presynaptic terminal plasma membrane, the release process is known as quantal release.
After a neurotransmitter diffuses across the synaptic cleft to the postsynaptic neuron, receptors embedded in the postsynaptic membrane bind to the neurotransmitter and cause an electrical or biochemical alteration in the postsynaptic cell. Excitatory signals cause the membrane to depolarize so that positive charge flows into the cell, and inhibitory signals cause the membrane to hyperpolarize, whereby positive charge flows out of the cell or negative charge flows into the cell. These electrical signals are integrated in the dendrites and cell body and, if depolarization is sufficient, an all-or-none action potential fires and transmits an electrical impulse along the axon (Chapter 2). Whether synaptic transmission is fast, occurring within several milliseconds, or slow—taking place in seconds or minutes—depends on the type of receptor targeted by the neurotransmitter 3–3.
3–3
Ligand-gated channels and G protein–coupled receptors. Ligand-gated ion channels, which mediate fast synaptic transmission, are composed of one or more subunits (eg, α β γ δ) embedded in the plasma membrane that form a central, gated pore. In response to the binding of transmitter, this type of receptor undergoes a conformational change, opening the gate and allowing ions to diffuse passively along concentration gradients through a hydrophilic opening in an otherwise hydrophobic bilayer. G protein–coupled receptors, which mediate slow synaptic transmission, transduce neurotransmitter signals through a different mechanism. These proteins do not form gated pores in the membrane; rather, the binding of transmitter induces a conformational change that allows the receptor to activate a heterotrimeric G protein (Chapter 4). The activated G protein dissociates into a free α subunit bound to GTP and a free βγ subunit dimer. Both can activate enzymes that synthesize second messengers; in addition, βγ dimers directly regulate certain ion channels. Second messengers also regulate ion channels, most often by activating protein kinases, which subsequently phosphorylate such channels (P). 1, 2, 3, cytoplasmic domains of G protein–coupled receptor; C, C-terminal tail of the receptor. (Adapted with permission from Kandel ER, Schwartz JH, Jessell TM. Principles of Neural Science, 4th ed. New York: McGraw-Hill; 2000:184.)

Fast synaptic transmission is mediated by transmitter- or ligand-gated ion channels, also called ionotropic receptors. The most extensively studied of these is the nicotinic acetylcholine receptor, which is composed of five subunits that enclose a central aqueous pore. When acetylcholine binds to this receptor, the pore opens transiently (1–10 ms) and allows the passage of approximately 20,000 positively charged Na+ ions. The vast majority of excitatory and inhibitory synapses in the brain utilize ionotropic receptors for the amino acids glutamate and GABA (Chapter 5). Molecular cloning has revealed that the basic design of most, if not all, ligand-gated channels is similar to that of voltage-gated channels (Chapters 2 and 5).
Many of the neurotransmitters involved in fast synaptic transmission also induce slower synaptic responses when they activate receptors coupled to heterotrimeric guanine nucleotide–binding proteins (G proteins). These receptors are often termed metabotropic receptors. Most other types of neurotransmitters, including the monoamines and neuropeptides, also activate G protein–coupled receptors. When a neurotransmitter binds to a G protein–coupled receptor, the receptor activates an associated G protein. The α subunit of the G protein subsequently dissociates from its βγ subunits, with both α and βγ subunits then activating downstream molecules, including specific types of ion channels (Chapter 4). These biochemical steps mediate slow synaptic transmission because they generally require more time to develop compared with the opening of a ligand-gated channel. Stimulation of G protein–coupled receptors does not always produce excitatory or inhibitory transmission; in some cases it modulates the actions of other neurotransmitters, such as glutamate.
In addition to regulating ion channels, G protein α and βγ subunits activate several enzymes that regulate the formation of second messengers such as cAMP, cGMP, and diacylglycerol (DAG). G proteins also regulate the flow of the second messenger Ca2+ into neurons. Once formed, second messengers stimulate or inhibit protein kinases or protein phosphatases, whose subsequent phosphorylation or dephosphorylation of ion channels results in the generation of postsynaptic electrical signals. Some second messengers, such as cAMP and cGMP, and G protein subunits (α and βγ) can directly bind to and modify the activity of specific ion channels. Moreover, these second messenger cascades regulate gene transcription and protein synthesis, which may alter the types and amounts of ion channels expressed by a neuron. Such activities can have long-lasting effects on neuronal function (Chapter 4). Thus, the involvement of G protein–coupled receptors greatly increases the complexity and flexibility of the types of electrical signals that can be generated by neurotransmitters.
Ligand-gated ion channels and G protein–coupled receptors each comprise multiple subtypes. A single neurotransmitter can activate a variety of these receptor subtypes, and several members of the same receptor family can colocalize at a single synapse; consequently, the signal received by a postsynaptic neuron can vary considerably and may be quite complex. Ultimately, the actions of any individual neurotransmitter are dictated by the receptor subtype to which it binds and by the subsynaptic localization of that receptor.
Before the advent of molecular cloning techniques, receptor subtypes were grouped according to their pharmacologic profiles, including their responses to particular agonists and antagonists. More recently, cloned receptors have been grouped more precisely on the basis of structural and functional similarities and according to their cellular localization. Some receptor subtypes tend to be located in particular cell types or subcellular regions. Presynaptic localization does not exclude postsynaptic localization, and the presence of some receptor subtypes—for example, the serotonin 5HT1A receptor—in both presynaptic terminals and postsynaptic cells further increases the complexity of synaptic communication. The activation of receptors located in the presynaptic bouton typically modifies the release of neurotransmitter. Presynaptic receptors that respond to the transmitter released by that terminal are called autoreceptors; presynaptic receptors that respond to other transmitters are sometimes called heteroreceptors. Variations in signaling pathways activated by different receptor subtypes underscore the central role of receptors in dictating the actions of the transmitters that bind to them.
Our current understanding of synaptic transmission is based on work performed in the 1950s, which uncovered the role of Ca2+ in neurotransmitter release at the neuromuscular junction (NMJ). The NMJ comprises a presynaptic motor nerve terminal and a postsynaptic specialization on the muscle cell known as an end plate. Although the NMJ differs significantly from a synapse in the CNS, it is similar enough to serve as a model. When the motor nerve of the NMJ is electrically stimulated, it releases a chemical message that produces an electrical signal in the muscle cell, called the excitatory postsynaptic current, which in turn causes contraction of the muscle. When this contractile process is paralyzed, the relationship between chemical transmission and the electrical response can be studied in isolation. The chemical transmitter at the vertebrate NMJ is acetylcholine, identified in 1921 as the first known chemical neurotransmitter.
Early studies of the NMJ found that the action potential per se was not required for acetylcholine release. Rather, the action potential caused the nerve terminal to depolarize, which in turn caused the release of transmitter. This was demonstrated by eliminating the action potential with tetrodotoxin, which blocks voltage-gated Na+ channels (Chapter 2), and changing the voltage in the nerve terminal with an electrode, which makes it possible to depolarize the terminal and induce transmitter release directly. It was subsequently discovered that eliminating extracellular Ca2+ prevented the release of neurotransmitter; it was therefore concluded that the entry of Ca2+ into the nerve terminal triggered transmitter release. We now know that the opening of voltage-gated Ca2+ channels in response to depolarization (Chapter 2) causes a rapid increase in the intracellular concentration of Ca2+, which in turn causes the release of neurotransmitter.
Related studies of the NMJ revealed that, in the absence of presynaptic stimulation, the postsynaptic cell exhibited small, spontaneous electrical responses. Equally important was the discovery that these miniature end plate potentials (MEPPs or minis) were all approximately the same size. Indeed, when the nerve was stimulated to evoke larger postsynaptic currents, the resulting end plate potentials were always integral sums of the MEPPs 3–4. These findings led to the hypothesis that a single MEPP represents the postsynaptic response to the smallest amount of transmitter released by a presynaptic cell, thus giving birth to the idea of quantal release.
3–4
Synaptic responses recorded during electrophysiologic experiments in the 1950s and 1960s. These experiments demonstrated that neurotransmitter is released from presynaptic terminals in discrete packages or quanta. Moreover, they determined that the postsynaptic response does not have a fixed amplitude but occurs in discrete steps, each of which represents the response to transmitter released from a single vesicle. A. Spontaneous miniature end plate potentials (S), which are electrical recordings of postsynaptic electrical activity in the absence of an external stimulus, and synaptic responses (end plate potentials) evoked by a weak stimulus. The recorded end plate potentials are generated by the release of one to four quanta of neurotransmitter; occasionally a weak stimulus fails to trigger such a release. (Reproduced with permission from Liley AW: The quantal components of the mammalian end-plate potential. J Physiol. 1956;133(3):571–587). B. A histogram that indicates the amplitude of the end plate potentials reveals peaks that are multiple integrals of the miniature end plate potentials (inset). (Reproduced with permission from Boyd IA, Martin AR: The end-plate potential in mammalian muscle. J Physiol. 1956;132(1):74–91.)

The precise physical equivalent of a quantum remained to be determined, but two pieces of evidence demonstrated that this elemental unit represented the transmitter contained in a single synaptic vesicle. First, electron micrographs revealed clusters of regular round vesicles lined up at the presynaptic membrane (see 3–2). Second, these vesicles sometimes appeared to be fused with the plasma membrane and, in the process of opening their lumen to the extracellular space, such vesicles look like the Greek letter Ω and are called omega profiles. Thus, small vesicles at the site of transmitter release appeared capable of releasing their contents into the synaptic cleft by fusing with the plasma membrane. Whether these synaptic vesicles contained neurotransmitter was unclear; however, biochemical studies demonstrated that synaptic vesicles at the NMJ contained acetylcholine. These findings validated the theory that a synaptic vesicle represents the anatomic equivalent of electrophysiologically defined quanta. Fittingly, the process of transmitter release was subsequently referred to as exocytosis.
Although morphologic studies indicate that there are tens to hundreds of synaptic vesicles in each nerve terminal, neurotransmitter is not released every time an action potential invades this structure. Instead, neurotransmitter release is a stochastic or probabilistic process: in response to an action potential it sometimes occurs and sometimes fails to occur. The probability of transmitter release can vary widely from synapse to synapse and can be modified by the activation of presynaptic neurotransmitter receptors, by drugs, and by recent synaptic activity.
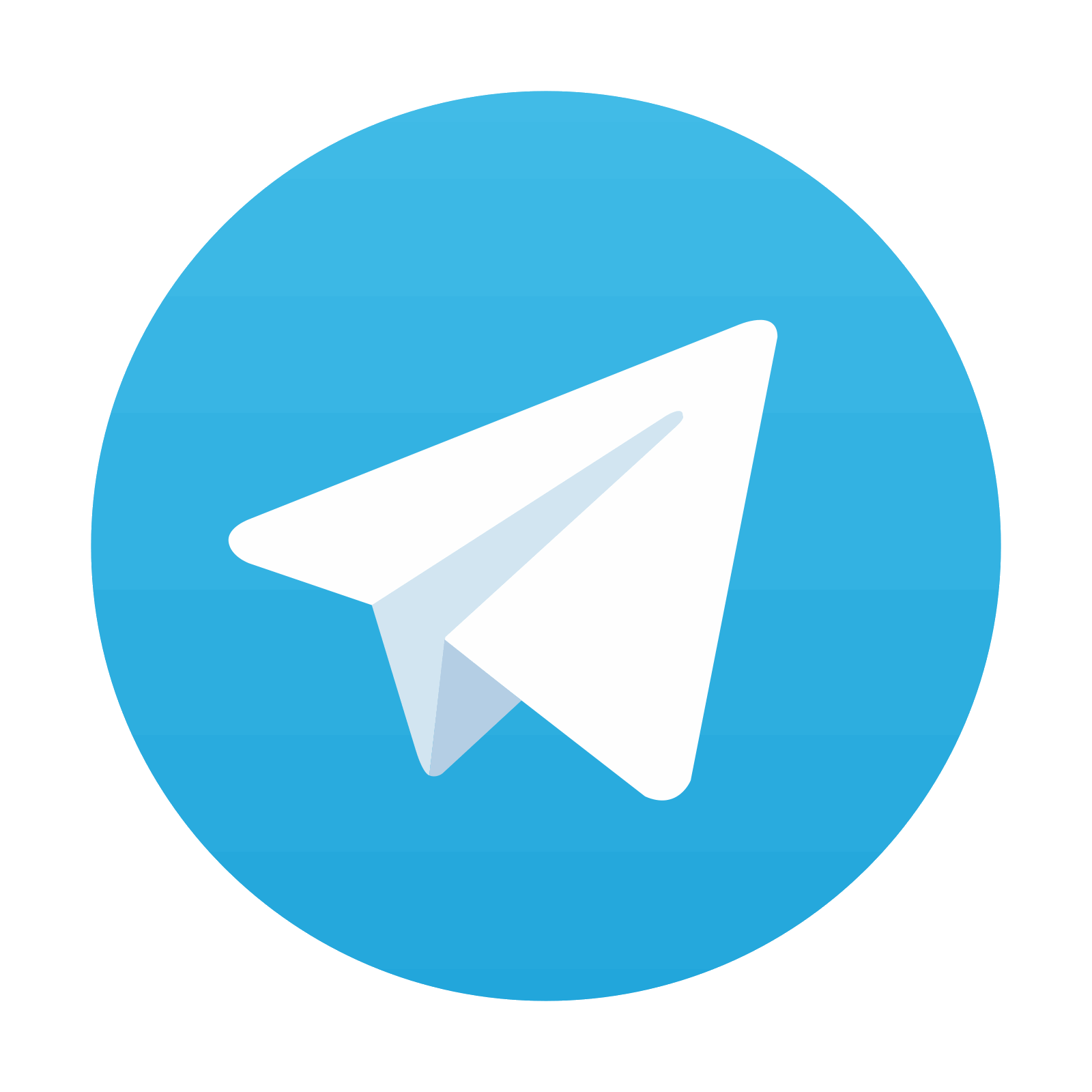
Stay updated, free articles. Join our Telegram channel
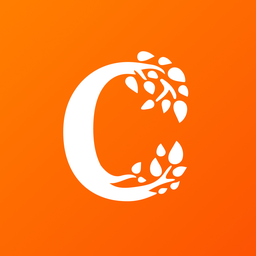
Full access? Get Clinical Tree
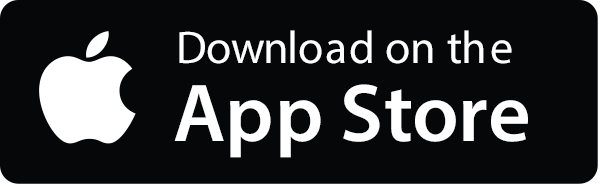
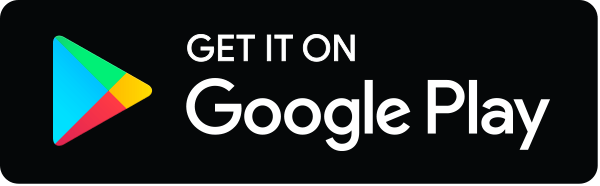