Plasma
ECF/ISF
ICF
CSF
Syrinxa
Sodium (mM)
135–145
138–142
5–15
135–154
145–150
Potassium (mM)
3.4–4.7
3.8–5
135–155
2.6–3.1
2.6–2.8
pH
7.35–7.45
7.44
7.3–7.6
Chloride (mM)
99–108
118
9.0
115–130
120–123
Calcium (mM)
2.1–2.6
1–1.5
1.05–1.7
Protein (g/L)
50–80
0.15–2
0.35–0.89
Glucose (mM)
3.9–6.1
2.8–4.2
3–6.1
Magnesium (mM)
0.7–1.0
1.0–2.3
1.09–2.1
Osmolality (mM)
280–296
280–296
280–296
280–296
280–296
Amino acids (g/L)
2.62
0.72
Most work done on CSF signalling pathways has concentrated on the cranial ventricular system, particularly around the third ventricle and hypothalamus. Neuroactive substances have been identified as being secreted from the pineal gland (melatonin), separately into the third ventricle (gonadotrophin-releasing hormone), as well as peptides (vasopressin and corticotrophin-releasing hormone) released from periventricular neurones and astrocytes (Redzic and Segal 2004; Veening and Barendregt 2010). They appear to have effects distant from the secreting site (Redzic and Segal 2004; Veening and Barendregt 2010). The influence of these neuroactive substances on spinal structures and any link with syrinx pathogenesis is not well established, although a major role in syrinx formation is unlikely. A role in pain production, contribution to neuronal loss, or as a potential molecular biomarker or therapeutic target is more feasible. The role of the specialised ependymal subtype, the tanycyte, in this process is discussed below.
17.2.2 Syrinx Fluid
A number of researchers have compared the fluid in a syrinx with that in the CSF, although most have concentrated on the protein concentrations (Freeman 1959; Schlesinger et al. 1981; J Wong 2012, personal communication). The electrolyte composition of the syrinx fluid has only rarely been examined and in very limited numbers, but it appears that syrinx fluid has a similar electrolyte composition to CSF (Table 17.1). This similar composition, combined with the lack of an anatomical barrier to fluid flow across syrinx and pial surfaces and the observation that tracer studies show rapid flow between the subarachnoid and interstitial spaces, via perivascular spaces , has been used to support a CSF origin for syrinx fluid (Brodbelt 2003; Cserr and Knopf 1992; Davson and Segal 1996; Foldi 1999; Kida et al. 1995; Rosenberg 1990; Weller 1998).
Syrinx fluid has been reported as having different protein content from that of CSF or plasma, and this may have implications for syrinx pathogenesis (According to J Wong MBBS, written communication, April 2012). The observed degree of difference may be influenced by whether the CSF is taken from the lumbar cistern, rather than adjacent to the syrinx. The protein content in CSF increases caudally along the spinal canal, ranging from 15 mg/100 mL in the ventricle to 36 mg/100 mL in the cistern magna and 59 mg/100 mL in the lumbar theca (Davson and Segal 1996). Eighty percent of CSF protein originates from the blood, whilst the other 20 % originates from neurones, glia and leptomeningeal cells within the central nervous system (Veening and Barendregt 2010). In Cavalier King Charles Spaniels, a comparative study of CSF in animals with a syrinx showed a higher protein and cell content, as compared to those with a Chiari and no syrinx (Whittaker et al. 2011). This difference in CSF content may be related to syrinx-induced cell damage and an inflammatory response in these animals. From the published literature, the protein content of syrinx fluid may be slightly higher than CSF, except in some post-traumatic cases where this difference is much greater (Laha et al. 1975; Nurick et al. 1970; Rossier et al. 1985, J Wong 2012, personal communication).
Protein could affect fluid flow into a syrinx by promoting diffusion. Diffusion describes the movement of a substance (solute or solvent) from a higher to a lower concentration (Elkinton and Donowski 1955). Osmosis , in contrast, is fluid movement down a concentration gradient established by the restraint of solute to one area, by either a semipermeable membrane or a continuously active transport process moving solute in one direction (Elkinton and Donowski 1955). Raised protein could produce a diffusion gradient in favour of fluid flow into a post-traumatic syrinx. If the gradient is great enough, significant pressures could develop. Although studies using CSF tracers suggest the volume is too great and velocity too rapid for diffusion alone to be a sufficient explanation, in some post-traumatic syrinxes diffusion along a protein-produced diffusion gradient might contribute to fluid ingress into the cavity (Rennels et al. 1990; Stoodley et al. 1996, 1999).
Although much has been published regarding the makeup of plasma and CSF protein compartments, there is nothing published on the specific proteins and amino acids that are present in syrinx fluid. These proteins may have implications in syrinx initiation, development or maintenance. Many of the proteins found in the serum are also found within the CSF (Davson and Segal 1996). These include hormones, interleukins , endorphins and various proteins used as biomarkers for some neurological conditions (Davson and Segal 1996; Hu et al. 2012a). Beta-2 transferrin is the desialated isoform of the iron-binding glycoprotein transferrin and is only found in CSF, perilymph and ocular fluids (Papadea and Schlosser 2005). It is not clear if beta-2 transferrin is found in syrinx fluid.
CSF biomarkers for multiple sclerosis are well recognised, although newer biomarkers for Alzheimer, frontotemporal lobar degeneration and Parkinson’s disease are being developed (Weller et al. 1998). As we understand the biochemical processes underlying the pathogenesis of syringomyelia, new biomarkers in the CSF or serum for syrinx propagation or development may occur. These could be related to inflammatory processes, oedema or water transport and might, in the future, help tailor drug treatment to syringomyelia subtype or pathological effect.
17.3 Tissue Biochemistry
The influence of the chemical processes within and around the cells surrounding a syrinx depends in part on the unique anatomy of the spinal cord. Tight junctions limiting fluid movement, compartmentalisation and extended cellular processes can all influence function. The following discussion will focus on the biochemical processes occurring in an anatomically orientated manner.
17.3.1 Fluid Barriers
Barriers exist between plasma and interstitial fluid (ISF) (the blood-brain barrier or BBB), between plasma and CSF (the blood-CSF barrier or BCSFB), between interstitial and intracellular fluids (ICF) and within the cord parenchyma (Abbott 2004; Leonhardt and Desaga 1975). A leaky barrier exists between the CSF and extracellular spaces, allowing mixing of interstitial fluid and CSF (Davson and Segal 1996; Del Bigio 1995). Barriers can consist of cell membranes, tight junctions between cells and various cellular transport processes that control solute and water movement. These transport processes include the aquaporins , pinocytic vesicles and a multitude of ion transport channels (see below) (Brodbelt and Stoodley 2007; Davson and Segal 1996).
The term blood-brain barrier refers to the anatomical and physiological complex that controls the movement of substances from the general extracellular fluid of the body into the extracellular fluid of the brain (Nolte 1999). In capillary endothelium , this manifests as tight junctions between adjacent cells and absence of pinocytic vesicles (Nolte 1999; Rosenberg 1990). Instead, interstitial fluid of the central nervous system is formed by an active process at the cerebral capillary. Interstitial fluid of the CNS also exchanges freely with CSF (Rosenberg 1990).
The blood-brain barrier is also functionally dynamic, with a wide permeability range, dependent on intra- and intercellular signalling events amongst endothelial cells, astrocytes, pericytes and neurones (Bonkowski et al. 2011; Neuwelt et al. 2011). The neurovascular unit , composed of pre- and postsynaptic endings, their related glia, pericytes and the capillary bed, engages in multiple signalling processes (Neuwelt et al. 2011) (Fig. 17.1). Ten to fifteen percent of all proteins that make up the neurovascular unit are transporters (Enerson and Drewes 2006). This highly complex unit has been examined in a post-traumatic rat model of syringomyelia. The presence of endothelial barrier antigen and loss of functional integrity, as assessed by extravasation of intravascular horseradish peroxidase, found a prolonged structural and functional disruption of the blood-spinal cord barrier in this animal model (Hemley et al. 2009). Astrocytes may play an active role in maintaining disturbed neurovascular unit function (Neuwelt et al. 2011). This disturbed blood-spinal cord barrier may contribute to fluid ingress into a syrinx (Hemley et al. 2009).
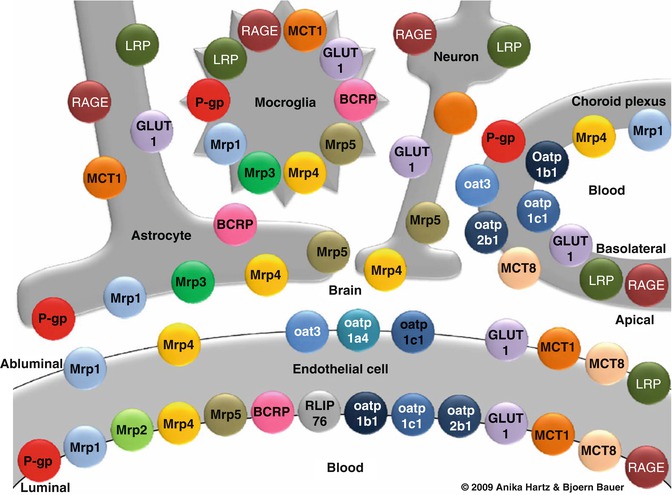
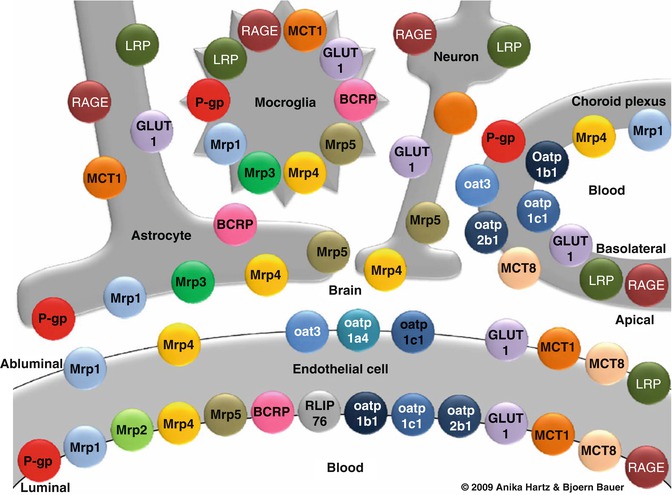
Fig. 17.1
Primary transporters in the neurovascular unit . The cellular relationships of carrier-mediated transport systems at the blood-brain interface, illustrating the complexity of the system which has a role in spinal cord fluid homeostasis. BCRP breast cancer resistance protein, GLUT1 glucose transporter-1, LRP low-density lipoprotein receptor-related protein, MCT monocarboxylic acid transporter, Mrp multidrug resistance protein, Oat organic anion transporter, Oatp organic anion transporting protein, P-gp P-glycoprotein, RAGE receptor for advanced glycation end products, RLIP76 Ral-binding protein-1 (Taken with permission from Neuwelt 2011)
Directly under the ependyma and between ependymal cells , there are basement membranes which form labyrinths, connecting the ependymal basement membrane with perivascular basement membranes of subependymal capillaries and postcapillary veins (Leonhardt and Desaga 1975; Cifuentes et al. 1992). These glycolipid and glycoprotein structures can hold fluid by swelling but may also function to form a pathway for movement of fluid and solutes, between the ependyma and subependymal vessels (Leonhardt and Desaga 1975).
17.3.2 The Central Canal, Ependyma and Tanycytes
The central canal of the spinal cord is lined by a continuous layer of ependymal cells and occasional tanycytes. Ependyma is a single-layered, cuboidal to columnar, ciliated epithelium, derived from germinal matrix cells (Bruni and Reddy 1987; Del Bigio 1995). In the brain, ependymal cells are connected by zonula adherens and have abundant gap junctions (Brightman and Reese 1969; Del Bigio 1995). They may function to regulate water movement, act as a barrier between the CSF and extracellular spaces and act as a niche of latent neural stem cells (Del Bigio 1995; Hamilton et al. 2009).
Tanycytes are specialised forms of ependymal cells, found in the ependymal lining or subependyma. They have radially directed basal processes that extend into the neuropil, where they typically enwrap blood vessels (Bruni 1998; Honda et al. 1999; Del Bigio 1995). The function of tanycytes in the spinal cord is unknown although neuronal guidance, structural support, neuroendocrine roles and a limited transport system, from the CSF to the ECF, have been suggested (Bruni 1998; Honda et al. 1999; Del Bigio 1995). One study looked at nestin 1 expression as a marker of latent neural stem cell properties in the ependymal cells of the central canal and found that this correlated with tanycyte morphology (Hamilton et al. 2009). Tanycytes implanted into a rat spinal cord injury model supported the regeneration of lesioned axons (Leonhardt and Desaga 1975). The functions of ependyma and tanycytes in human spinal cord may be less important than in the brain but may still play a role after cellular destruction in the spinal cord and provide an interesting avenue for further investigation.
In two large human autopsy series, central canal stenosis or occlusion was present in 75–97 % of cases after the third decade, and isolated segments occurred between stenotic regions (Aboulker 1979; Milhorat et al. 1994; Yasui et al. 1999). Stenosis has been postulated as occurring because of mild ependymal injury or viral infection (Del Bigio 1995; Milhorat et al. 1994). The stenotic sections of the central canal so formed have been postulated to act as an initial site for fluid build-up into a cyst (Brodbelt 2003). As well as their potential in treatment as neural stem cells, ependymal cells and tanycytes may also have a role in providing an initial cavity, an isolated segment of the central canal which, in some cases with the correct filling mechanism, will lead to syrinx formation.
17.3.3 Astrocytes
Adjacent to the ependyma is the spinal cord parenchyma, composed mainly of astrocytes, which are the most numerous cell type in the central nervous system. Astrocytes have a number of functions and are important in many biochemical mechanisms within the spinal cord. They support neurones by supplying some citric acid cycle intermediates, taking up excess neurotransmitter and maintaining stable glutathione levels (Sidoryk-Wegrzynowicz et al. 2011). Astrocytes also regulate the extracellular environment including acid base balance, potassium buffering and free radical scavenging (Sidoryk-Wegrzynowicz et al. 2011). They produce and release growth factors, including transforming growth factor β, platelet-derived growth factor and nerve growth factor (Sidoryk-Wegrzynowicz et al. 2011). There are dynamic and reciprocal signalling networks between astrocytes and neurones (Sidoryk-Wegrzynowicz et al. 2011). Other areas of astrocytic function of interest in patients with a syrinx include the removal of excess neurotransmitters and the role of aquaporins . Astrocytes are also implicated in the pathogenesis of neuropathic pain (for more detail see Chap. 16). Any or all of these functions may be affected in patients with a syrinx, and the role of the astrocyte must be viewed as pivotal in this area.
17.3.4 Neurotransmitters
Glutamate is the main excitatory neurotransmitter in the brain. Glutamate is formed by the reductive amination of the Krebs cycle intermediate, α-ketoglutarate. It acts on metabotropic and ionotropic receptors (Ganong 1995). Metabotropic receptors are serpentine G-protein-coupled receptors that are excitatory and neuromodulatory in action (Ganong 1995; Watkins 2000). Ionotropic receptors are excitatory ligand-gated ion channels subdivided by selective agonist action into kainic acid, AMPA (2-amino-3-(5-methyl-3-oxo-1,2- oxazol-4-yl)propanoic acid) and N-methyl-D-aspartate (NMDA) receptors (Ganong 1995). In other words, kainic acid , AMPA and N-methyl-D-aspartate are all substances used to selectively stimulate subtypes of ionotropic glutamate receptor. Kainic acid and AMPA channels are involved in fast synaptic transmission and permit Na+ influx and K+ efflux when open, whilst N-methyl-D-aspartate allows Ca2+ passage and is normally blocked by Mg2+ (Watkins 2000). Quisqualic acid is an agonist for AMPA and group I metabotropic receptors that act via intracellular inositol triphosphate (Watkins 2000). In excess, glutamate and some of the substances used experimentally to stimulate glutamate receptor subtypes, including quisqualic acid, can cause selective neuronal death via AMPA and metabotropic receptor activation, leading to excessive free intracellular calcium. In this way it is ‘excitotoxic’ (Ganong 1995).
Debridement of the necrotic area following spinal cord injury in rats and dogs improves recovery, suggesting that ongoing biochemical processes occur after the initial injury (Freeman and Wright 1953). One group of candidates for such a role is the excitatory amino acids . Excitatory amino acids are released after both spinal cord injury and ischaemia and lead to glutamate receptor activation, calcium influx and selective neuronal cell death (Liu 1991; Marsala et al. 1994; Panter 1990; Simpson et al. 1990; Tator 1991; Urca and Urca 1990; Urushitani et al. 1998). The amount of excitatory amino acid released correlates with the degree of trauma, and one study suggests that this release may be the direct result of neuronal activity (Liu et al. 1991; Panter et al. 1990). Quisqualic acid (an excitatory amino acid) and kainate appear to be more neurotoxic than N-methyl-D-aspartate, and selective antagonism of these substances confers some neuroprotective effects (Li and Tator 2000; Urca and Urca 1990). In fact, intraspinal injection of quisqualic acid produces extracanalicular syrinxes (Brodbelt et al. 2003a, b; Yang et al. 2001; Yezierski et al. 1993). Combined with kaolin in the subarachnoid space to produce arachnoiditis, the cavities so produced enlarge over time and are histologically identical to that seen in humans (Brodbelt et al. 2003a, b). Other candidates potentially involved in secondary cord damage include proteolytic enzyme release, liberation of free fatty acids, free radical production, phospholipid degradation and abnormalities of prostaglandin production (Edgar and Quail 1994; Kao and Chang 1977; Reddy et al. 1989).
Fifty to ninety percent of adult patients with syringomyelia will have pain (Todor et al. 2000). Substance P and calcitonin gene-related peptide have both been implicated in pain production. In a human pathological study, substance P distribution was decreased both around and cranial to a syrinx cavity but increased caudally (Todor et al. 2000). A recent study in Cavalier King Charles Spaniels also showed an altered distribution (Hu et al. 2012a). It is not yet clear how this alteration relates to the pain patients feel or if other excitatory amino acids or neurotransmitters, such as GABA, also play a part, but successful identification of the role of such chemicals could lead to better treatment.
17.3.5 Aquaporins
Much interest has been generated regarding the transmembrane group of water channels, known as aquaporins (AQPs) (Fig. 17.2). In normal human spinal cord, aquaporin subtype 4 (AQP-4) is seen on all astrocytes (Nesic et al. 2010). AQP-1 is found on the apical surface of the choroid plexus epithelium and sensory axons in the dorsal horn, AQP-8 is found in the ependymal cells around the central canal , and AQP-9 is found in neurones and astrocytes (Badaut 2009; Gunnarson et al. 2004; Kimelberg 2004; Oshio et al. 2004). AQP-4 functions to enable fast water influx or efflux, driven by osmotic or pressure gradients (Solenov et al. 2002; Yang et al. 2008). AQP-4 has a role in normal ion and water homeostasis in the spinal cord and may contribute to the pathophysiological response in disease states.
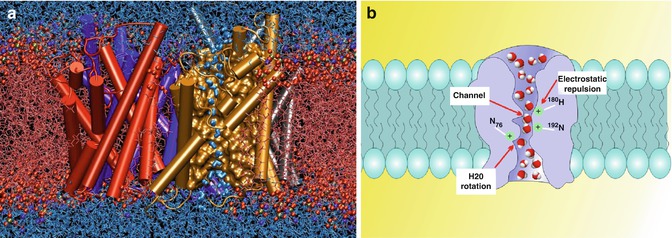
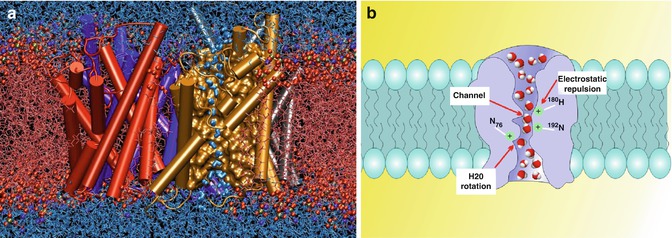
Fig. 17.2
Aquaporins have a role in tissue fluid homeostasis although their role in syringomyelia is inconclusive. (a) A snapshot of an atomic simulation in progress. Boomerang-shaped water molecules slip as they march single file through the narrow pore of the gold aquaporin , whilst the red balls and fibres that make up the cell’s membrane keep the outside water (top) from mixing with the cellular pool (bottom) (Taken with permission from Emad Tajkhorshid, Klaus Schulten, Theoretical and Computational Biophysics Group, University of Illinois at Urbana-Champaign, 2004 Winner of Visualization Challenge in Science and Engineering, organised by the National Science Foundation and Science Magazine). (b) Schematic depiction of water movement through the narrow selectivity filter of the aquaporin channel (Taken with permission from Opossum58 at the German language Wikipedia)
Following injury, AQP-4 expression changes, and this change is both site and time dependent. In a series of three spinal cord-injured patients, pathological examination of the injured spinal cords demonstrated no AQP-4 in GFAP2-labelled astrocytes surrounding the damaged area, whilst astrocytes in the spared white matter rim had intense AQP-4 labelling (Nesic et al. 2010). Similar combinations of astrocytes with AQP-4 absence and AQP-4 overexpression have been reported in animal models of spinal cord injury and post-traumatic syringomyelia (Aghayev et al. 2011; Nesic et al. 2010, A Watling 2003, personal communication). Furthermore, it appears that the AQP-4-negative cells either change to or are replaced over time by AQP-4-overexpressing cells. This change in AQP-4 expression is delayed in more severe injury, and this delay has been suggested to be inversely related to the likelihood of motor and sensory recovery in rat models (Nesic et al. 2010).
Tissue hypoxia alters AQP-4 expression. Hypoxia is described following trauma or in the tissue around the syrinx and may be due to pressure effects (Brodbelt 2003; Milhorat et al. 1996). One effect of tissue hypoxia is the release of hypoxia-induced factor 1a 3 which appears to upregulate AQP-4 (Ding et al. 2009). This mechanism leading to cytotoxic oedema may be protective. Hypoxia-induced cellular energy depletion alters ion transport mechanisms, changes the normal ion balance and leads to a build-up of sodium within the neurone (Malek et al. 2003). To maintain cellular osmolality and electriconeutrality, potassium and chloride ions leave the cell (Malek et al. 2003). This tenfold increase in extracellular potassium and chloride is taken up by the astrocytes (Blank and Kirshner 1977; Malek et al. 2003). Upregulation of AQP-4 allows water rapidly to follow the ions and may be seen as a mechanism to maintain normal cellular osmolality (Nesic et al. 2010).
The early post-injury phase of AQP-4-negative astrocytes may contribute to the accumulation of vasogenic oedema by slowing the process of fluid removal around the area affected and working against the aquaporin homeostatic process (Zador et al. 2009). The overall effect of AQP-4-driven astrocytic swelling on functional recovery after spinal cord injury may depend on the balance between vasogenic oedema, a protective astrocyte role in removing extracellular ions and the harmful effects of astrocytic swelling (Nesic et al. 2010).
Despite this evolving interest, there has been variable support for a direct role of aquaporins in syringomyelia pathogenesis. Using a kaolin model of syringomyelia in the rat, both Aghayev et al. and Hemley et al. found no significant difference in AQP-4 expression between controls and syrinx-induced animals (Aghayev et al. 2011; Hemley et al. 2012). However, in a similar rat model, Zhang et al. demonstrated a relationship in AQP-4 expression proportional to the degree of central canal expansion (Zhang et al. 2012). Nesci et al. found no correlation in a rat post-traumatic syrinx model, between AQP-4 protein levels and syrinx size or enlargement over time (Nesic et al. 2010).
Our understanding of AQP-4 in tissue water homeostasis is developing. Aquaporins remain an area of interest as regards our understanding of syrinx pathogenesis and the functional alterations in the surrounding spinal cord that can lead to pain and disability. Further study of aquaporins may, in the future, lead to new treatments.
17.3.6 The Arterial Circulation
Tissue homeostasis also depends on the relationship of the arterial and venous structures within the spinal cord. In humans, there is a pial network (the vasocorona) of small arteries interconnecting anterior, posterolateral and posterior spinal arteries of the cord. These give off peripheral (centripetal) arteries which supply mainly white matter tracts (Kiernan 1998). The anterior spinal arteries send central (sulcal) arteries into the ventromedian fissure before turning laterally and supplying, alternately, right or left central grey matter (Thron 1988). A study of 13 rats, using a vascular corrosion cast technique, suggested that ventral and ventrolateral pial arterial plexuses do not exist and that a correspondingly greater proportion of the blood supply to the white matter comes from branches of the central (sulcal) arteries (Koyanagi et al. 1993). Humans have large numbers of small peripheral penetrating branches, approximately 100 times the number of central arteries. These terminate in the external fibre tracts or the marginal zone of the grey matter (Thron 1988). The relationship between the arterial supply and the astrocytes and blood-brain barrier has been discussed above. There is, currently, an enormous body of work looking at control and regulation of vascular supply of the spinal cord, yet relatively little has been carried out in patients with syringomyelia. Although fluid movement in the perivascular space has been proposed and modelled, altering electrolyte and protein balances or water channel flow across the neurovascular junction could all change the balance of fluid flow and has not yet been investigated (Bilston et al. 2003; Brodbelt et al. 2003a).
17.3.7 The Venous Circulation
Venous drainage of the spinal cord is by a series of irregular plexiform channels, inconsistent in size and position (Thron 1988). The most consistent and continuous veins are the anterior median spinal vein, running deep to the anterior spinal artery, and the posterior median spinal vein running in the dorsal midline (Thron 1988). There are other longitudinal channels that run along the anterior and dorsal rootlets (Kiernan 1998; Thron 1988). Sulcal veins are associated with sulcal arteries in the ventromedian fissure and drain the central grey and medial part of the anterior white matter into the anterior spinal vein mainly in the lumbosacral area (Koyanagi et al. 1993; Thron 1988). Radial veins are more important in the thoracic and cervical regions, draining the anterior and lateral white columns and significant amounts of adjacent grey matter into superficial venous channels (Koyanagi et al. 1993; Thron 1988). Posterior medial septal and oblique veins drain the posterior columns into posterior spinal veins (Koyanagi et al. 1993).
The relationship of the venous structures to overall fluid outflow from the spinal cord has been a matter for discussion (Brodbelt 2003). If fluid is entering the syrinx from perivascular spaces , there is no watertight lining to the cavity and this is a dynamic situation, then where does the fluid go? A recent large animal model attempted to look at fluid outflow and found that it occurred in a diffuse manner, into the surrounding extracellular space and towards the central canal and perivascular spaces (Wong et al. 2012). The precise relationship with the venous anatomy, if there is one, is not clear.
17.4 Pathological Processes
A number of pathological processes have been studied in relation to syringomyelia, and early theories included inflammation, ischaemia or interstitial oedema as the direct cause of a syrinx. Although, no longer thought to be directly causing the enlarging fluid-filled cavity, many of these processes are seen adjacent to a syrinx and may contribute to the growth, maintenance or symptoms generated by the syrinx.
17.4.1 The Inflammatory Response
The inflammatory responses of the nervous system to trauma result in oedema and sometimes in cyst formation (Fischbein et al. 1999; Guizar-Sahagun et al. 1994; Kakulas 1999; Tator and Fehlings 1991; Zhang et al. 1997). Descriptions of the histological features of the spinal cord following trauma were initially based on animal work but have since found agreement with human pathological studies and can be subdivided into three stages: necrosis, repair and stability (Guizar-Sahagun et al. 1994; Squier and Lehr 1994). From 1 to 14 days after injury to the spinal cord, parenchymatous haemorrhage, vascular thrombosis, oedema, axonal segmentation and inflammatory infiltrates are seen (Guizar-Sahagun et al. 1994; Kakulas 1987; Tator and Fehlings 1991; Wagner et al. 1978; Zhang et al. 1997). The repair stage occurs between 2 and 15 weeks after injury, when revascularisation occurs and macrophages phagocytose necrotic tissue, before then disappearing, leaving cavities within the cord (Guizar-Sahagun et al. 1994; Wagner et al. 1978; Williams 1980). Finally, a stage of stability occurs, between 15 weeks and 1 year after trauma, when the lesioned area has become cystic. The cysts may have trabeculae and may be collapsed with a sparse surrounding chronic inflammatory infiltrate and arachnoiditis (Guizar-Sahagun et al. 1994; Kakulas 1987; Tator and Fehlings 1991; Wagner et al. 1978).
Chronic inflammation or infection has been proposed as causative mechanisms in patients with meningitis who develop syrinxes (Charcot and Joffroy 1869; Klekamp 2002) (Fig. 17.3). Infection, it was proposed, could cause a syrinx either from an induction of an inflammatory response or by causing ischaemia (Charcot and Joffroy 1869; Klekamp 2002). Milhorat et al. suggested that viral infections could induce central canal occlusion and demonstrated that suckling hamsters , infected with reovirus, produced a similar pathological appearance to that seen clinically in human adults (Brodbelt et al. 2005; Milhorat and Kotzen 1994; Milhorat et al. 1994). Isolated segments of the central canal so formed could provide an isolated cavity promoting a noncommunicating canalicular syrinx. However, many conditions that predispose to syrinx formation are not associated with inflammation or infection. Inflammation, therefore, probably contributes to syrinx pathogenesis in some cases by leading to arachnoid adhesion formation and the hydrodynamic effects that this produces, rather than by acting as the main pathogenic mechanism. Treatment aimed at stopping or reducing adhesion formation by targeting inflammatory processes, especially after injury or localised infection, could act to reduce the risk of syrinx formation.
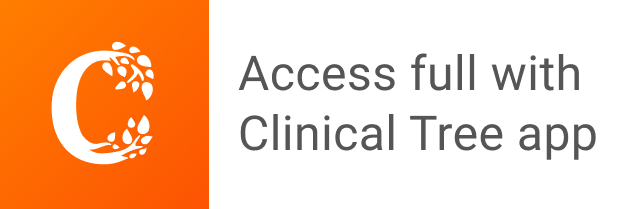