Introduction
Down syndrome (DS) is the most common genetic cause of intellectual disability, affecting approximately 16 per 10,000 live births in the United States [1–3] (see Chapter 1). DS is linked to trisomy of chromosome 21 (HSA21), which contains between 200 and 300 genes that influence the phenotype of people with DS including a high incidence of gastrointestinal and cardiac abnormalities (see Chapter 11, and a select list of genes is listed in Table 1 and a full list of genes can be found in Refs. [15, 16]). Improvements in medical care along with social enrichment have led to a significant extension in lifespan and increased quality of life; however, it has led to an increase in the prevalence of Alzheimer’s disease (AD) [17–19] (see Chapter 1). AD is the leading cause of dementia in the general population and is characterized by progressive loss of cognition and daily functions. There are two hallmark pathologies required for an AD diagnosis: amyloid plaques, composed of aggregated amyloid-beta (Aβ) peptides; and neurofibrillary tangles (NFT), composed of hyperphosphorylated tau [20].
Table 1
Gene | Protein | Function | Reference |
---|---|---|---|
ADAMTS1 | ADAM metalloproteinase with thrombospondin type 1 motif, 1 | Secreted protease known to be induced by IL-1β | [4] |
ADAMTS5 | ADAM metalloproteinase with thrombospondin type 1 motif, 5 | Secreted protease known to be induced by IL-1β and TGFβ | [5] |
CBS | Cystathionine-beta-synthase | Production of hydrogen sulfide (H2S); a regulator of inflammation | [6] |
CXADR | Coxsackie virus and adenovirus receptor | Activation of JNK and p38-MAPK pathways leading to production of pro-inflammatory cytokines | [7] |
IFNAR1 | Interferon (alpha, beta, and omega) receptor 1 | Activates JAK/STAT mediated pathway in response to IFNα/β | [8] |
IFNAR2 | Interferon (alpha, beta, and omega) receptor 2 | Activates JAK/STAT mediated pathway in response to IFNα/β | [9] |
IFNGR2 | Interferon gamma receptor 2 | Activates JAK/STAT mediated pathway in response to IFNγ | [9] |
PRMT2 | Protein arginine methyltransferase 2 | Blocks the actions of NF-κB in the nucleus | [10] |
RIPK4 | Receptor-interacting serine-threonine kinase 4 | Necessary for signaling through TNFR1 | [11] |
S100β | S100 calcium binding protein B | Constitutive expression by astrocytes, released in response to TNFα | [12] |
SOD1 | Super oxide dismutase 1 | Scavenges superoxide radicals producing H2O2 and O2 | [13] |
TIAM1 | T-cell lymphoma invasion and metastasis 1 | Necessary for cytokine-mediated generation of oxidative species through NDAPH oxidase | [14] |
In people with DS, overexpression of Aβ begins prenatally due to the extra copy of the amyloid precursor protein (APP) on chromosome 21 (see Chapter 10), with progressive deposition of senile plaques (SP) usually after 30 years of age [21] (see Chapter 2). Virtually, all people with full trisomy 21 have sufficient senile plaques and neurofibrillary tangles for a neuropathological diagnosis of AD by 40 years of age [22–25]. While people with DS have AD pathology by midlife, the onset of dementia is often many years later. The mean age for dementia diagnosis is 55 years, but almost 90% of this population will develop dementia by 65 years of age [26, 27] (see Chapters 13 and 15).
Neuroinflammation in Alzheimer’s disease
Neuroinflammation is a major contributor to the development of neurodegenerative disorders, and a crucial factor in the onset, progression, and pathogenesis of Alzheimer’s disease. Inflammatory processes are multidimensional and multifaceted in the brain, involving many different mediators and cells that can be both pro- or antiinflammatory. While other cells in the brain contribute to the neuroinflammatory response, we will focus on microglia for the purposes of this chapter as dysfunction in these cells is critically implicated in the pathomechanisms of neurodegenerative disorders involving inflammation.
Glial cells, which include astrocytes, microglia, and oligodendrocytes, influence the homeostasis and function of the central nervous system throughout life by involvement with several important mechanisms. Astrocytes can clear neurotransmitters at the synapse, release transmitters, and regulate ion balance [28–30]. In addition, microglia are also in direct contact with other cells and blood vessels. They are critical for proper neuronal function in the brain by regulating synaptic pruning and synaptogenesis, programmed cell death, and engulfing cellular debris [31–34].
Microglia cells were originally described in the 1920s in the human brain prior to injury as “resting cells” [35, 36]. After injury, microglia change their morphology in a process called hypertrophy, associated with a shortening of the cellular processes and the enlargement of the nucleus. The ability to stain and image these cells contributed greatly to our understanding of their behavior and function. Microglia are identified through their expression of several classic immune activation markers such as Iba1 (ionized calcium binding adaptor molecule 1 is a microglia/macrophage-specific calcium-binding protein), MHC-II (associated with antigen presentation), CD68 (a lysosomal protein), and CD36 (a class B scavenger receptor). More recently, CD163 (a heme scavenger receptor) was found to be increased in AD suggesting the presence of a unique disease-associated cell population [37]. All of these markers are highly localized and are present on microglia surrounding Aβ plaques and NFTs [38]. Microglia have been implicated in the neuropathology of AD as well as having a role in the clearance of pathology. It is likely that both phenomena are at play in disease progression but at different times. Whether the response is toxic or beneficial seems to be dependent on the type, duration, and concentration of events stimulating inflammation. In order to understand neuroinflammatory processes, researchers have been examining cytokines, secreted by microglia, to better understand microglial contributions to inflammation and pathogenesis.
Cytokine levels, including interleukin 1β (IL-1β), interleukin-6 (IL-6), tumor necrosis factor α (TNF-α), and transforming growth factor β (TGF-β), are altered in AD. In 1995 Griffin et al. showed in human autopsy tissue that IL-1β is associated with the transition from diffuse amyloid deposits to neuritic amyloid plaques [39]. This idea was later expanded through the development of a “cytokine cycle” hypothesis suggesting that IL-1β is produced in response to amyloid deposits leading to a cascade of events. The cytokine cycle is associated with increased APP production and processing in neurons, recruitment, and activation of astrocytes, which in turn can lead to further increase in IL-1β production [40]. In cases of mild cognitive impairment (MCI—thought to be the earliest signs of AD), serum IL-1β was elevated, suggesting IL-1β could be a potential biomarker of conversion from MCI to AD [41]. Genome-wide association studies (GWAS) have identified polymorphisms in IL-1β associated with AD (reviewed in Ref. [42]). Further studies are needed to determine the actual impact of these polymorphisms with AD risk. In contrast to the detrimental role suggested by these early data on IL-1β, other studies suggest that in the hippocampus of the APPswe/PS1dE9 mouse model of AD, an overexpression of IL-1β can result in the reduction of amyloid pathology by up to 50% [43].
The major source of IL-6 in the brain is from microglia; however, astrocytes, neurons, and endothelial cells are also capable of releasing this cytokine that mediates both inflammatory reactions and immune responses [44–47]. In AD, IL-6 is elevated in pathologically relevant regions within the brain such as the cortex and hippocampus [48]. IL-6 has a myriad of pathological downstream effects such as induction of acute phase proteins (a class of proteins whose plasma concentrations change in response to inflammation), increased vascular permeability (which may lead to serum proteins leaking into the brain causing more inflammation), activation of lymphocytes (leading to the release of more cytokines), and others (reviewed in Ref. [49]). Conversely, IL-6 also has positive effects such as enhancing neuronal survival [50–52] and suppressing demyelination [53]. In a mouse model of AD, enhancement of IL-6 activity led to enhanced microglial phagocytosis of amyloid deposits and reduced plaque burden [54]. These studies suggest IL-6 has multiple effects in the brain and it is important to consider that both beneficial and adverse effects can occur.
TNF-α also has potent pro-inflammatory and cytotoxic consequences [55–57], with beneficial effects reported on hippocampal neurons [58, 59]. This dichotomous effect is mediated through actions of the TNF-α receptors, which include TNF-α receptor 1 (TNFR1) and TNF-α receptor 2 (TNFR2) [60]. TNFR1 is responsible for mediating neuronal death via caspase-8-activated apoptosis and TNF receptor-associated death domain protein (TRADD) [61, 62]. TNFR2 is believed to mediate the beneficial, pro-survival functions of TNF-α through the nuclear factor κB (NF-κB) mediated antiapoptotic pathways [63]. This is an abridged view of TNF-α actions and associated receptors. A more extensive description has been reviewed by Holbrook and colleagues [64]. In AD, TNFR1 expression is elevated in the brain whereas TNFR2 is decreased [61]. Clinical trials investigating the use of etanercept, which incorporates a fusion protein containing TNFR2 and the Fc portion of IgG, to mimic the beneficial effects of higher TNFR2 levels in the brain, are under evaluation in AD. Etanercept was previously used to treat Crohn’s disease and arthritis [65]. Preliminary studies showed peri-spinal delivery of etanercept improves cognition in a small number of patients with mild to moderate dementia [66–68]. However, the trial was discontinued, mainly due to the small number of participants, which could have affected the randomization of participants in the study. Even though subcutaneous etanercept was well tolerated, a large and more heterogeneous group should be tested before confirming its effects on cognition [68].
Finally, TGF-β is a growth factor important for tissue development, homeostasis, and repair [69]. TGF-β is unlike other cytokines that have been discussed as it is primarily associated with repair mechanisms but it is not with damaging or toxic effects in the brain. TGF-β is most frequently associated with the formation of a glial scar in the brain [70] and upregulation of extracellular matrix proteins [71–73]. TGF-β levels in AD are increased in the brain [74] while decreased in the serum [17]. In AD brain, lower levels of TGF-β receptor protein are associated with more extensive AD neuropathology [75].
Characterizing the inflammatory state in the brain, especially in the presence of AD pathology or other neurodegenerative diseases, is an important area of research. Microglia in the brain are described as having two states: “classical activation” and “alternative activation” [76]. While this classification of microglia has been modified since 2006, the basic principles still remain. Classical activation includes pro-inflammatory cytokines such as IFNγ, IL-1β, TNFα, and IL-6. Alternative activation was used to describe states associated with antiinflammation, repair, and wound healing all mediated by IL-10, TGFβ, IL-4, IL-13, arginase 1 (AG1), and tissue remodeling factors found in Inflammatory Zone 1 (FIZZ1) and chitinase 3-like 3 (YM1) [76]. Additionally, studies using human autopsy tissue show similar mixed pro- and antiinflammatory phenotype that could help with understanding disease progression in patients [77].
Neuroinflammation in Down syndrome—Genetics
Many inflammatory pathways described for AD in this chapter are observed with aging and AD in DS. Interestingly, triplication of chromosome 21 results in the extra copy of many genes that may play a role in AD and dementia, in addition to APP. Examination of chromosome 21 reveals genes that are associated with inflammatory and immune processes. Table 1 describes the genes that are triplicated on chromosome 21 that are known to have immune function and roles in inflammation and we will discuss each of these genes and potential impact of the effect (some of these genes are also described in Chapter 10) (Fig. 1).

We now turn to a discussion of several individual genes in Table 1. Of the ADAMTS family (a disintegrin and metalloproteinase with thrombospondin motif), two members are located on chromosome 21 and are triplicated in DS: ADAMTS1 and ADAMTS5. ADAMTS1 and ADAMTS5 act as proteinases involved in degrading extracellular matrix proteoglycans, such as aggrecans and versicans [78, 79]. Additionally, ADAMTS1 contains a signal peptide in the N-terminal region which suggests secretion from the cell [80]. ADAMTS1 and ADAMTS5 are induced by IL-1β, indicating they are both dependent on inflammatory responses [5, 6]. In DS, ADAMST1 has a fivefold increase in protein expression, whereas ADAMST5 does not show a significant change in expression level [81]. Due to the induction by IL-1β, it could be hypothesized that the triplication of ADAMST1 and ADAMST5 may lead to an exacerbated degradation of extracellular matrix proteins in response to any inflammatory insult. To expand on this hypothesis, Griffin et.al in 1989 showed that the brains of people with DS have a higher level of IL-1β immunoreactivity indicating there is more IL-1β present potentially leading to more stimulation of ADAMTS [82].
Cystathionine beta synthase (CBS) is an enzyme present in the cytosol responsible for the conversion of homocysteine. One of the alternate reactions catalyzed by CBS leads to the production of hydrogen sulfide (H2S). CBS binds to NO or CO in its heme pocket which allows for the activation of the enzyme and production of H2S [83]. Since its discovery, H2S has become recognized as an atypical cellular messenger with many cellular functions [84] and as a complicated signaling molecule. H2S has an apparent bimodal action in inflammation. Specifically, research suggests that low levels of H2S have an antiinflammatory effect, whereas high levels may exacerbate inflammation. Several extensive reviews discuss this bimodal effect of H2S (see Refs. [85, 86]). More studies are needed to investigate the overexpression of CBS, subsequent increase in H2S, and its potential role on AD pathology in DS. Additionally, understanding whether the amount of H2S in DS patients elicits an antiinflammatory or pro-inflammatory phenotype is of particular interest for potentially targeting CBS.
CXADR is a gene encoding for a protein called coxsackie virus and adenovirus receptor (CXADR). CXADR works as both an adhesion molecule associated with tight junctions and as a viral receptor. It is highly expressed in the brain as well as many secretory organs such as the pancreas, testis, and small intestines [87]. In the heart, an increase in CXADR is observed in models of myocardial inflammation and cardiac injury, suggesting an innate role of CXADR in the inflammatory response [88]. Additionally, CXADR can induce stress-activated mitogen-activated protein kinase (MAPK) pathways leading to an increase in the production of interferon-γ (IFNγ), IL-12, IL-1β, TNFα, and IL-6 [4]. In addition to this inflammatory response, CXADR also plays an important role in tight junctions, which in endothelial cells helps facilitate transendothelial migration of neutrophils from the blood into the brain [89]. If the expression of CXARD is altered in endothelial cells within the cerebrovasculature of people with DS, there may be an altered infiltration of peripheral immune cells in the brain impacting the innate inflammatory response.
Of the interferon receptor family, IFNAR1, IFNAR2, and IFNGR2 are all triplicated in most cases of DS [90]. IFNAR1 and INFAR2 respond to IFNα, IFNβ, or IFNο. Upon ligand binding, there is an activation of the JAK/STAT signaling pathway which leads to the induction and production of pro-inflammatory gene expressions such as IL-1β, TNFα, and IL-6. INFGR2 follows the same signaling cascade as before, however it responds specifically to IFNγ. A mouse model of DS, with trisomy 16, shows triplication of both IFNGR2 and INFAR2. These mice rarely survive to birth due to the significant level of pathology in utero. In this model, anti-IFN IgG treatment to the fetuses improves survival and trisomy phenotype [91]. In cultured neurons from the DS mouse model, a partial knockout of either IFNAR2 or INFGR2 leads to improved growth and viability of neurons [92]. Due to the triplication of these genes in DS, there is a likely hyperreactive response to IFN in DS that could potentially lead to an increased inflammatory response, both in the brain and systemically.
Protein arginine methyltransferase 2 (PRMT2) is an enzyme that catalyzes the methylation of arginine. Arginine methylation is also a major regulator of the JAK-STAT pathway [93]. Additionally, degradation of proteins containing methylated arginine can occur naturally and result in the production of asymmetric dimethylarginine (ADMA) [94]. ADMA is an inhibitor of nitric oxide synthase (NOS), which is a key player in normal cellular signaling and inflammation [95]. People with DS with pulmonary hypertension have increased expression of ADMA compared to neurotypical controls with pulmonary hypertension; however, it remains unclear if triplication of PRMT2 results in these changes [96]. If PRMT2 was the reason for this increase, one could hypothesize that there would be a decrease in NO production and an activation of JAK-STAT pathway, both inducing an inflammatory state in DS.
Receptor-interacting serine-threonine kinase 4 (RIPK4) is a protein kinase important for multiple cell signaling pathways. These pathways include RIPK4 activating NF-κB [97] as well as being involved in the signaling cascade of the TNFα receptor TNFR1 [11]. Of interest, TNFR1 is implicated in the toxic effects of TNFα and one could hypothesize that the overexpression of RIPK4 may increase responsiveness of TNFR1 to TNF-α, exacerbating the effects of TNFR1. However, more research on this topic in DS is necessary.
S100β is a protein primarily located in the brain and expressed by astrocytes. In response to IL-1β and cyclic-AMP, it is secreted by the astrocytes and can lead to dichotomous inflammatory actions [98]. In low concentrations, S100β appears to enhance the survival of neurons [99] as well as stimulate neurite outgrowth [100]. However, in high concentrations S100β increases cell death [101] and causes apoptosis [102]. Overall these studies suggest that the overexpression of S100β in the brains of people with DS may contribute to pathological aging.
T-cell lymphoma invasion and metastasis 1 (TIAM1) is a quinine nucleotide exchange factor for Rac1 [103]. The activation of Rac1 is necessary for activation of NADPH oxidase [104]. TIAM1 has a critical role in cytokine induction of NADPH oxidase through IL-1β [7]. These conclusions were based upon studies in pancreatic β-cells; however, one could hypothesize that in DS, an overexpression of TIAM1 may lead to an increase in oxidative stress involving IL-1β. Supporting this hypothesis, TIAM1 expression is increased in fetal DS brains compared to control [105].
In summary, there are a significant number of genes present on chromosome 21 related to inflammation, which could have significant impact on neuroinflammation throughout the lifespan of people with DS, contributing to brain development, aging, and AD neuropathology.
Neuroinflammation in Down syndrome: Biomarker studies
The search for biomarkers of AD in DS has accelerated due to longitudinal studies of aging. Chapters 6 and 7 provide detailed descriptions of the outcomes of fluid biomarkers for AD in DS and Chapters 8 and 9 describe neuroimaging biomarkers that may reflect neuroinflammation. The focus here will be on the results of autopsy studies where brain tissues donated by people with DS were examined.
Neuroinflammation as a function of age and AD: Autopsy studies
Given the upregulation of multiple genes associated with inflammation and immune function in DS, one might predict there may be neuroinflammation with aging and that this would be exacerbated with the development of AD in DS. Further, cerebrovascular pathology described in Chapter 4 may also be a significant contributor to neuroinflammation in DS. The presence of significant cerebral amyloid angiopathy (CAA) observed by neuroimaging and autopsy studies shows significant CAA and microhemorrhages, supporting the hypothesis that serum proteins may leak into the brain and lead to neuroinflammation. Examination of autopsy tissue, neuroimaging, and fluid biomarker in people with DS points to a significant and potentially unique neuroinflammatory signature. Neuroinflammation is associated with aging and with AD neuropathology in DS. Complement system activation, increased protein levels of S100β, cytokine release, and changes in microglial cell morphology are some of the known inflammatory mechanisms occurring in the brains of individuals with DS.
The complement pathway is made of proteins that work to eliminate pathogens and stimulate inflammation to fight infection [106]. Complement activation occurs in AD brain (reviewed in Ref. [107]) and also appears active in response to AD pathology in DS. For example, C1q, the first protein in the classical complement pathway that leads to inflammation, is increased in the brains of people with DS with AD neuropathology [108, 109].
Microglial cells, key mediators of inflammation in the brain, show interesting morphological changes in DS suggesting degeneration of these cells in association with increased NFT accumulation [110]. Indeed, a more recent study shows that not only are there fewer resting microglial cells (surveilling their environment) with age in DS but there are also increased numbers of dystrophic microglial cells, which may reflect dysfunction or death [111]. Similarly, increased dystrophic and rod-like microglia cells are also observed in adults with DS and AD. The analysis of cytokine expression across the lifespan of individuals with DS demonstrates that important inflammatory mediators are exacerbated before the occurrence of overt AD pathology and appear to decrease at advanced stages. Cytokine expression in adults with DS is region specific. Both the hippocampus and frontal cortex show elevated levels of IL-1β, IL-6, IL-15, eotaxin-3, monocyte chemoattractant protein-1 (MCP-1), macrophage inflammatory protein-beta (MIP-1β), and the thymus- and activation-regulated chemokine (TARC); however, the chemoattractants IP-10 and MDC were higher in the frontal cortex only, while increased IL-1α and IL-4 were observed only in the hippocampus [112]. It is important to mention that the evaluation of inflammatory markers in the plasma demonstrates that high levels of TNF-α, IFN-γ, IL-6, IL-8, and IL-10 are only observed in people with DS showing no symptoms of AD. This finding suggests that there is not only a characteristic inflammatory phenotype in DS but that AD pathology develops silently over many years [113]. In a previous study of DS autopsy cases with a range of ages, RNA levels of several key neuroinflammatory proteins were quantified and revealed a distinct profile in the DS brain with AD neuropathology [114]. CD86 and FCGR1β were uniquely increased only in individuals with DS with AD, indicating immune complexes may be forming in the brain that can activate microglial cells and toll-like receptors [114]. In contrast, increased CD86 and FCGR1β RNA expression has been observed only rarely in sporadic AD.
In transgenic mouse models of AD where microglial cells are selectively eliminated through the use of PLX5622, Aβ concentrations tend to shift in distribution to deposit within the blood vessels of the brain (CAA—see Chapter 4) [115]. Higher levels of CAA are typically observed in the aging DS brain, thus in addition to the overproduction of Aβ, it is possible that dying microglial cells are contributing in part to this form of cerebrovascular pathology. Indeed, AD patients with CAA have a distinct clearance of Aβ that is mediated by microglial CD11b binding to extracellular C3b/Aβ complexes, which distinguishes them from AD patients without CAA or controls. This interaction between microglial CD11b and C3b/Aβ complexes delivers both active C3b and Aβ to the microvasculature, and leads to a late activation of the complement system [116]. Potentially, this shift in the microglial binding of Aβ, associated with the morphological changes of microglia, could also help explain the high levels of CAA in DS.
More compelling evidence of a role for microglia and inflammation in AD pathogenesis has evolved from studies showing that variants in the triggering receptor expressed on myeloid cells 2 (TREM2), which is also implicated in microglial phagocytosis, are associated with an increased risk of developing AD [117]. Through sequential protease cleavage, the full-length TREM2 produces soluble TREM2 (sTREM2) [118] and recent evidence suggests that it possesses a neuroprotective function [119]; however, its physiological function remains elusive. In AD patients, higher sTREM2 in the CSF is associated with attenuated cognitive decline [120]. Very little is known of the role of TREM2 in DS with AD. There are descriptions of elevated TREM2 and sTREM2 levels in young adults with DS [121] and declining levels in the frontal cortex with age [122], but no reports describing the influence of the receptor directly on the phenotype and/or function of microglial cells. Most importantly, with varying levels of sTREM2 throughout different stages of aging and AD, it is likely that TREM2 might also have divergent effects on microglia in the brains of people with DS and AD.
Another inflammation-associated molecule that may be important for people with DS is S100β, as described previously in this chapter. The gene for S100β is located on chromosome 21 and is elevated throughout the lives of individuals with DS [40], with twice the number of astrocytes expressing S100β in people with DS at all ages compared to controls. While S100β is vital for growth and development of the central nervous system, elevated levels have significant negative consequences. Overexpression of S100β causes abnormal neuronal growth [100, 123] and is commonly found in astrocytes associated with Aβ plaques [82, 124]. Transgenic mice that overexpress S100β have increased dendritic density in the hippocampus when young, but the density of the dendrites drastically decrease at 1 year of age [125]. Similarly, fetuses with DS initially have normal dendritic morphology, but dendritic development becomes stunted in infants with DS [126, 127]. This decrease in dendrites corresponds well with learning and memory deficits, suggesting that S100β may play a significant role in the progression of cognitive decline in aging individuals with DS.
Neuroinflammation is a major contributor to the development of AD and other neurodegenerative disorders [128–130]. Autopsy studies provide significant advances in our understanding of immune and inflammatory pathways in people with DS, demonstrating a unique pattern of signaling pathways and cellular response to AD pathology.
Drug development for AD with a focus on neuroinflammation
The inflammatory component of AD has been a key target for drug development in the potential treatment for AD, and immunotherapy has been one interesting approach. This form of treatment was first demonstrated in 1999 [131] using either an active or passive immunization approach to treat patients through an increase in anti-Aβ antibodies (reviewed in Ref. [132]). Immunization for Aβ leads to a reduction in Aβ in the brain in transgenic mouse models of AD and is associated with improvements in memory and learning [133, 134]. Injection of anti-Aβ antibodies into the brains of transgenic mouse models shows that the removal of Aβ was dependent upon microglial activation [135, 136]. Further, systemic administration of anti-Aβ antibodies leads to a transient activation of microglia [135].
Administration of nonsteroidal antiinflammatory drugs (NSAIDs) has been another approach to target the inflammatory responses. While NSAIDs initially showed great promise through a retrospective epidemiological study [137], a prospective clinical trial through the NIA/NIH, called the ADAPT trial, failed to show benefits of long-term NSAID use [138]. The potential pathways NSAIDs target make the story more complicated. Some NSAIDs modify the γ-secretase enzyme that cleaves APP to form Aβ, which can lead to the production of more Aβ38 instead of the more toxic Aβ40 or Aβ42 [139] but these specific NSAIDs were not used in the ADAPT trial. However, a subset of patients in the trial did end up benefiting from NSAID use. Specifically, a subgroup of people with AD who received Naproxen showed attenuated cognitive decline termed as “slow-decliners,” whereas cognitive decline was accelerated in those categorized as “fast-decliners” [140]. While it remains unclear why these groups progressed differently, these results suggest that differing inflammatory states exist between various AD cohorts leading to some patients benefiting from NSAIDs and others not. Future studies are needed to validate this idea.
The unique neuroinflammatory profile in aging adults with DS might also serve as a cautionary note when considering immunotherapeutics as this treatment can lead to elevated CD86 and FCGR1β, exacerbating inflammation in DS, which could lead to adverse events [141]. Thus clinical trials intended to modify neuroinflammation in the brains of people with DS will require some careful and dedicated clinical trials (see Chapter 16).
Conclusions
Genes found on chromosome 21 and therefore triplicated in DS have a wide range of functions but there are many associated with pro-inflammatory responses. Throughout this chapter we have discussed each of the genes triplicated in DS, and are summarized in Table 1. In brief, the triplication of major interferon receptors, IFNAR1, IFNAR2, and IFNGR2, suggests an enhanced interferon signaling. This enhanced signaling in turn could lead to an increased production of pro-inflammatory mediators such as IL-1β, TNFα, and IL-6. These cytokines are associated with oxidative stress and with the triplication of TIAM1, SOD1, and PRMT2 will lead to an even greater exacerbation of oxidative stress. TIAM1 enhances oxidation through NADPH oxidase, SOD1 at high levels enhances oxidation, and PRMT2 inhibits nitric oxide production. We have also discussed the role of neuroinflammation in AD, the potential implications in DS, and potential biomarkers for AD in people with DS. These combined factors likely lead to the enhanced neurodegeneration in DS in response to primary pathologies such as Aβ plaques and neurofibrillary tangles.
Markers of neuroinflammation may also serve as biomarkers, as described in Chapters 6 and 7. The study of late onset sporadic AD can provide us with significant information on the role of neuroinflammation in pathogenesis, but more is needed to understand specific aspects of inflammation in DS. The triplication of many inflammatory-associated genes in DS leads to a unique inflammatory environment worthy of study. In turn, expanding our knowledge of inflammation in DS will not only be critical for AD in DS but also for AD in the general population.
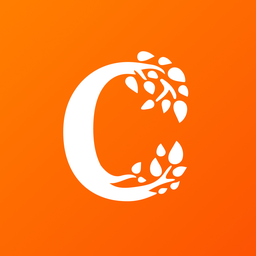
Full access? Get Clinical Tree
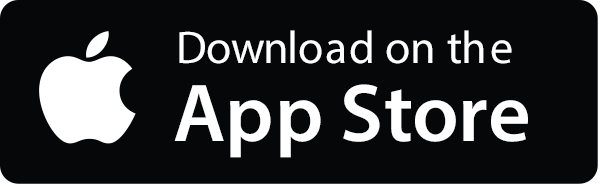
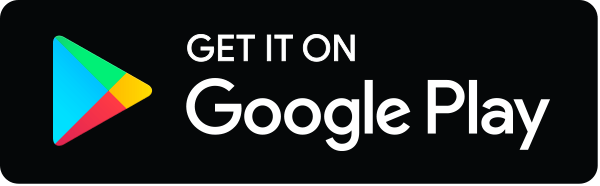