Genetics of oxidative stress in Down syndrome
Growing studies support the hypothesis that oxidative stress (OS) contributes to neurodevelopmental defects, neuronal dysfunction, as well as the accelerated aging phenotype of people with DS [1–3]. OS is a condition that results from either overproduction of reactive oxygen and nitrogen species (ROS/RNS), or by reduced antioxidant response or both. The central nervous system (CNS) contains high levels of fatty acids that, together with high aerobic metabolic activity, are responsible of the brain susceptibility to undergo oxidative damage [4–6]. ROS such as superoxide anion (O2−), hydrogen peroxide (H2O2), and hydroxyl radical (HO
) are produced as by-products of aerobic respiration and various other catabolic and anabolic processes [7]. The major source of free radicals is the mitochondrial oxidative phosphorylation pathway (OXOPHOS), in which electron leakage causes the formation of O2−
that, in turn, is converted by mitochondrial-resident MnSOD into H2O2 and O2 [8, 9]. Indeed, dysfunction of complex I has been demonstrated to be one of the major processes responsible for overproduction of ROS in skin fibroblast from people with DS, isolated from both fetal and adult subjects [10].
Several studies have recently shown that OS results directly from triplication of some Hsa21 genes together with the effects related to a deregulation of gene/protein expression associated with the trisomy [11]. Increased production of ROS is also associated with mitochondrial dysfunction, which occurs in DS cells as early as from embryonic life [12]. A number of reports showed OS markers in DS phenotype [13–15], although a direct cause-and-effect relationship between the accumulation of oxidative damage and clinical manifestation of DS has not yet been clarified. Growing evidence supports the idea that OS is a chronic feature in DS brain that initiates postnatally, even during embryonic development, and further progresses with aging. This feature represents a strong risk factor for subsequent neurodegeneration in aged people with DS [16, 17].
In order to understand the causes of OS, the first insights can be obtained by mapping Hsa21 on which a number of genes such as SOD1, APP, BACH1, ETS2, CR, S100B, among others, are believed to be involved in the increased OS levels found in DS individuals and in the Ts65Dn mouse model thereof [18]. Among trisomic genes, one of the most relevant as a potential OS inducer is copper-zinc superoxide dismutase (SOD1). The enzyme represents the first line of antioxidant defense by catalyzing the dismutation of O2− to molecular oxygen (O2) and H2O2, which can be converted by catalase (CAT) and by (selenium-containing) glutathione peroxidase (GPX) to water [19]. However, the triplication of Hsa21 leads to an imbalance in the ratio of SOD-1 to CAT and GPX, resulting in the accumulation of H2O2 [20]. Interestingly, all DS tissues, in addition to the brain, have own altered SOD-1/GPX activity ratio [4] that likely explains a condition in which an accumulation of H2O2 and its by-products occurs. Expression levels of SOD-1 were found to be approximately 50% higher than normal in a variety of DS cells and tissues, including erythrocytes, B and T lymphocytes, and fibroblasts. In addition, a decreased expression of peroxiredoxin 2 was detected in DS fetal brain which may contribute to enhanced susceptibility of DS neurons to free radical damage [21].
Interestingly, overexpression of SOD seems to trigger mitochondrial dysfunction. Shin et al. demonstrated that transgenic mice overexpressing wild-type human SOD1 (Tg-SOD1) display several mitochondrial defects including mitochondrial swelling, vacuolization, and that these features are also associated with learning and memory deficits [6]. Mitochondrial ATP synthase alpha/beta chain and elongation factor Tu protein levels were altered in Tg-SOD1, while no changes in the levels of antioxidant proteins were found. These changes may indicate synaptosomal damage and neuronal loss in Tg-SOD1 hippocampus that can ultimately result in cognitive defects in DS.
Studies demonstrated that loss of mitochondrial structure and function associated with increased ROS production contributes to the majority of DS pathological phenotypes [22]. This is not only the case of DS but also other neurodevelopmental disorders, such as Rett’s syndrome and autism [23, 24], as well as it occurs in neurodegenerative diseases, including Alzheimer’s and Parkinson’s diseases [25].
Busciglio and colleagues showed that neurons in the fetal brains of DS exhibited a significant increase in intracellular ROS which is paralleled by elevated lipid peroxidation (LPO) [12, 26]. In addition, a proteomic study reported that OS in fetal DS is mainly caused by reduction of antioxidant enzymes involved in removal of hydrogen peroxide, such as glutathione transferases and thioredoxin peroxidases, then from SOD-1 itself [27]. DS mitochondria are characterized by reduced ability to produce ATP through oxidative phosphorylation (OXPHOS), decreased respiratory capacity, and disruption of mitochondrial membrane potential, all associated with loss of mitochondrial dynamics. These mitochondrial defects are present in all DS cell types, from peripheral tissues to the brain [28]. Thus mitochondrial dysfunction is considered an inherent feature of DS, associated with a condition of increased OS [29].
Elevated levels of OS could also be caused by increased production of amyloid beta-peptide (Aβ), the cleavage product of APP. Several studies have extensively demonstrated that both Aβ(1–40/42) are able to induce OS. Butterfield’s group and others [14, 18, 30] proposed that Aβ(1–42) inserts as oligomers in the lipid bilayer and serves as a source of ROS thereby initiating lipid peroxidation (LPO) [31]. Deposition of senile plaques is observed in postmortem brain from aged DS individuals [32] and levels of both Aβ(1–42) and Aβ(1–40) in plasma are higher in DS compared with non-DS controls [33] (see also Chapter 2). In addition, Aβ plays a role in the modulation of metal homeostasis through coordination of metal ions—Zn2 +, Cu2 +, and Fe2 +—that participate in both production of and defense against ROS and are required to regulate neuronal activity in synapses and other biological functions in the brain. Furthermore, studies from Anandatheerthavarada et al. [34] pointed out that full-length APP itself may have toxic effects, in particular, damaging mitochondria. The concept is that in the presence of increased APP expression levels, a progressive accumulation of transmembrane-arrested APP impaired mitochondrial function, which in turn resulted in disturbance of energy metabolism. Moreover, mice overexpressing wild-type human APP show cognitive defects and neuronal pathology similar to what was observed in AD models, though these mice do not show significant Aβ deposition in the hippocampus [35]. Nonetheless, APP processing was nonamyloidogenic, but increased levels of phosphorylated tau were observed. These findings support the notion that trisomy of APP may promote mitochondrial dysfunction in DS independently from Aβ deposition.
Results from our group and others also suggest the involvement of BACH1, encoded on Hsa21, in the regulation of the antioxidant response in DS [5, 36]. BACH1 is a transcription repressor that acts as a key regulator of the expression of genes involved in the cell stress response [37]. In physiological conditions, BACH1 is assembled in the form of a heterodimer with small Maf proteins, which bind the antioxidant response elements (AREs) of DNA, thus negatively regulating the expression of specific target proteins. When OS levels are elevated, the function of BACH1 is suppressed by promoting BACH1 nuclear export and thereby enhancing the expression of its gene targets. For example, when intracellular heme levels rise, as may occur under a pro-oxidant insult, nuclear BACH1 binds heme and dissociates from the AREs thus allowing the transcription of genes such as quinone oxidoreductase-1 (NQO1), glutathione S-transferase (GST), glutamate-cysteine ligase (GCL), and heme oxygenase-1 (HO-1). In DS, it is likely that upregulation of BACH1 could block the induction of antioxidant genes, therefore promoting increased OS in the cell [5].
By mapping Hsa21, another candidate gene that is related to OS is the enzyme carbonyl reductase (CBR). Carbonyls, which are toxic metabolic intermediates, are detoxified by either oxidation performed by aldehyde dehydrogenase (ALDH) or by reduction to their corresponding alcohols by carbonyl reductase (CBR) and/or alcohol dehydrogenase (ADH). Protein levels of both these enzymes were found to be increased in several brain regions of DS and AD patients because of enzyme induction by elevated carbonyls in DS and AD [38].
Crosstalk between OS and proteostasis
OS-mediated damage to proteins is particularly important in aging and age-related neurodegenerative diseases. In the majority of cases, OS-induced posttranslational modifications cause nonreversible modifications of protein structure that in turn results in its impaired function. Generally, oxidation of proteins could affect different processes, including protein expression, protein turnover, cell signaling, eventually leading to cell death [39, 40]. Another major consequence of protein oxidation is the formation of protein aggregates, which are often toxic to cells if allowed to accumulate. Insoluble aggregates can be formed as a result of covalent crosslinks among peptide chains, as in the case of Aβ and hyperphosphorylated tau (p-Tau) in AD or by OS-mediated carbonylation of hydrophobic amino acids.
It is well known that the age-dependent accumulation of aberrant proteins results from both the increased occurrence of damage and the decreased efficiency of the protein quality control system (PQC) [41–43]. The PQC comprises three main systems that are responsible for regulating protein homeostasis: unfolded protein response (UPR), ubiquitin-proteasome system (UPS), and autophagy [44]. Several studies have shown that ROS are able to target components of the PQC, leading to loss of their functions [45]. In addition, upon oxidative modifications, proteins undergo several structural changes that are not always correctly recognized by the proteasome, thus generating impaired protein function [43, 45]. The balance between functional proteins, present in young/healthy cells, and damaged or altered proteins, present at higher concentration in aged/diseased cells, depends mainly on their modification and turnover.
Published studies from our laboratories identified several oxidatively modified proteins in DS brain, prior to and after development of AD [4, 46]. Among the proteins identified by redox proteomics to be oxidatively modified, either by increased carbonylation or HNE modification, we found proteins involved in several intracellular processes such as: (i) neuronal trafficking, (ii) the proteostasis network, (iii) energy metabolism, and (iv) mitochondrial function [4]. Intriguingly, all of these processes directly or indirectly rely on ATP consumption to occur efficiently. Reduced ATP levels, increased ROS, impaired calcium homeostasis, and altered mitochondrial permeability are characteristic mitochondrial defects of degenerating neurons in many neurodegenerative disorders.
Further, redox proteomics studies performed on human brains from people with DS and AD demonstrated that a number of oxidized proteins are common between the two pathologies, namely: GRP78, UCH-L1, HSC71, and GFAP. Among the components of the PQC, GRP78, UCH-L1, cathepsin D, V0-ATPase, and GFAP, were increasingly carbonylated in the frontal cortex in DS at about 20 years of age compared with their age-matched controls [4, 46]. These initial findings suggest that younger cases with DS may already show disturbance of the proteostasis network possibly linked to increased OS, many years before frank appearance of AD neuropathology.
Clearance of oxidatively modified proteins most often occurs through the proteasome system. The proteasome is the principal pathway to remove damaged proteins, and intact proteasome function is essential to preserve cellular homeostasis during OS. Moreover, protein aggregates and cross-linked proteins are able to bind to the proteasome, which makes the degradation of other misfolded and damaged proteins less efficient. Accordingly, levels of oxidized proteins in AD are associated with loss of the activity of the 20S proteasome [47]. Further, studies from the Davies and Grune laboratories showed that moderately oxidized proteins are preferentially recognized and degraded by the proteasome, while severely oxidized proteins cannot be easily degraded and, instead, inhibit the proteasome [42, 48].
In addition to the proteasome, autophagy plays a crucial role in the removal of toxic/aggregated proteins and impaired organelles that could damage cells during stress. Autophagy was initially considered as a nonselective degradation pathway in response to nutrient deprivation. More recent studies highlight that autophagy is a key process to regulate intracellular homeostasis in nonstarved cells by degrading cargo materials such as aggregated proteins, damaged mitochondria, and other organelles as well as extracellular pathogens [49, 50]. Most late-onset neurodegenerative disorders share defects in autophagy associated with the presence of intraneuronal aggregates of misfolded proteins, which are substrates for autophagic degradation [51]. However, the autophagy pathway is complex, with multiple steps and nodes of regulation and this makes identifying critical perturbations in the pathway quite difficult. Further, the absolute contribution of autophagy dysfunction to disease progression has yet to be established. Given that many neurodegenerative diseases are late-onset, it is possible that small alterations in the turnover of proteins will have cumulative effects that manifest later in life [49]. A master regulator of autophagy is mTOR [52] and the role of mTOR signaling in DS and AD is discussed later. We suggest that chronic exposure to OS leads to the impairment of both UPS and the autophagy-lysosome system, involved in the removal of toxic protein aggregates [22].
Primer of the mTOR pathway
The mammalian (aka mechanistic) target of rapamycin (mTOR) is a serine/threonine protein kinase of 289 kDa that belonging to the phosphatidylinositol 3-kinase-related kinase protein (PIKK) family [53]. mTOR is a ubiquitously expressed protein, mainly cytoplasmic, involved in the modulation of processes involving cell proliferation, mortality, survival, and protein synthesis [54]. The phosphorylation of mTOR at Thr-2446, Ser-2448, and Ser-2481 within the kinase catalytic (KIN) domain is correlated with increased mTOR activity. Adjacent to the KIN domain is the FKBP12 rapamycin-binding domain (FRB), the site of inhibitory interaction between rapamycin and mTOR. The binding of rapamycin to FKBP12 disturbs the formation of the mTORC1, thus reducing mTOR activity [55]. mTOR is known to be part of two different protein complexes: mTORC1 and mTORC2, which differ in components, upstream and downstream signaling, and responsiveness to rapamycin. Both mTORC1 and mTORC2 share the catalytic mTOR subunit, the mammalian lethal sec-13 protein 8 (mLST8), the DEP domain containing mTOR-interacting protein (Deptor), and the Tti1/Tel2 complex [56]. Regulated and coordinated activities of mTORC1 and mTORC2 are necessary for normal development and maintenance of neurons and brain homeostasis [57, 58]. Depending on the phosphorylated residue, these phosphorylation events may culminate in either mTOR inhibition or activation [59].
Classical mTORC1 positive inputs consist of growth factors, hormones, chemokines, nutrients (e.g., glucose or amino acids), and cell energy status (ATP/AMP ratio) [60]. mTORC1 stimulation occurs mainly through the phosphoinositide PI3K/Akt signaling pathway. In response to ligand binding, by insulin or insulin growth factor (IGF-1), the activity of the receptors, IR and IGF1-R, is induced by auto-phosphorylation on multiple tyrosine residues. In succession IR and IGF1-R phosphorylate insulin receptor substrates 1–4 (IRS1–4) [61]. The phosphorylation of IRS is linked to PI3K activation through the recruitment and signaling of p85 and p110 subunits. PI3K is then involved in the transformation of phosphatidylinositol 4,5-bisphosphate (PIP2) to phosphatidylinositol 3,4,5-trisphosphate (PIP3). All of this pathway is negatively regulated by the inositol phosphatase and tensin homolog (PTEN) [58]. Increased PIP3 levels recruit Akt to the membrane, where it is activated by phosphorylation of Thr-308 and Ser-473 residues by activation of phosphoinositide-dependent kinase-1 (PDK-1), mTORC2, and DNA-PK. In turn, Akt phosphorylates and inhibits TSC2, a negative regulator of mTORC1 [62, 63].
The regulation of protein homeostasis (protein synthesis/degradation) is one of the principal functions of mTORC1 [61]. Active mTORC1 phosphorylates components of the protein synthesis machinery, including ribosomal S6 kinase 1 (S6K1), and the translation inhibitor eukaryotic translation initiation factor 4E-binding protein (4E-BP) [59]. S6K1 presents two different isoforms, the key one for consideration in this chapter being p70 (p70S6K), mainly resident in the cytoplasm [64]. Autophagy is a finely organized process throughout eukaryotic cells that can digest abounding organelles, macromolecules, and proteins, and reutilize their breakdown products. Such degradation pathway holds an important task in both the maintenance of basal intracellular turnover and in the cellular adaptation to a variety of stresses [65]. At the current state of knowledge, autophagy is commonly differentiated in three different pathways: microautophagy, macroautophagy, and chaperone-mediated autophagy (CMA). Among the three types of autophagy, macroautophagy is certainly the best characterized. Macroautophagy encompasses the process of degradation of cytoplasmic moieties, including organelles, that involves formation of a double membrane (autophagosome) surrounding the moieties to be degraded, ubiquitylation of the autophagosome, and transport to and fusion of its membrane with the lysosome to form an autophagolysosome from which contents are ejected to the lysosome for degradation. After the formation of the autophagolysosome, cargo degradation is entirely dependent on the lysosomal acidic environment, which is actively maintained through the V-ATPase-mediated proton gradient. At acidic conditions, lysosomal hydrolases such as cathepsin B, D, and L break down autophagic substrates, and the resulting small molecules are transported back to the cytosol for nutrient recycling [53].
Accumulating evidence suggests that mTOR is also able to sense mitochondrial activity and cellular energetic status [66]. This feedback of the mitochondria to mTOR and its downstream targets p70S6K1 and 4EBP1 may support a molecular link between growth signals and cellular metabolism [67, 68]. However, the molecular mechanisms associating mTOR with mitochondria are still under investigation. Intriguingly, mTOR was found to be linked with mitochondria, suggesting a direct physical interaction [66, 69]. mTORC1 regulates mitochondrial oxidative function by positively controlling the activity of PGC1-α (PPARγ coactivator 1), a nuclear cofactor that modulates mitochondrial biogenesis and oxidative metabolism [70]. Nonetheless, mitochondria and their physical dynamics play a fundamental part at several stages of autophagy from the initial assembly of the autophagosome to autophagy-mediated cell death [71]. Noting the importance of mitochondria in brain metabolism, defective mitochondria need to be removed, not only because they are inefficient in ATP production, but because free radical leak from such mitochondria elevate harmful ROS. The process of degradation of misshaped and defective mitochondria is mitophagy. This is a macroautophagic process associated with mitochondria specifically, involving a complex of the proteins PARKIN/PINK1 and other factors. Since mitophagy is a subset of macroautophagy, the remaining processes are the same in both mitophagy and macroautophagy as described before.
mTOR then becomes critically important in DS because if the mTORC1 complex is activated, macroautophagy and mitophagy are inhibited, permitting cellular debris to accumulate and oxidative damage to occur in neurons, ultimately leading to their death. Therefore means of inhibiting activation of mTORC1 conceivably could be important in improving the quality of life of DS people.
mTOR as a link between Aβ and tau pathology
mTOR is expressed at high levels in the brain, primarily in neurons. During embryonic development, mTOR signaling functions as a potent neuronal survival and division signal responding to growth factors and guidance hints, including IGF-1 and insulin. Though the precise mechanisms are still not fully comprehended, regulated and coordinated activities of mTORC1 and mTORC2 are necessary for normal development of neurons and brain [57, 58]. The mTOR pathway supports extension of neurites (dendrites and axons) in brain development [72]. In terms of dendritic morphogenesis, increased activation of mTOR results in increased dendrite branching, higher numbers of immature filopodia-like protrusions in the dendrites, and a decrease in the density of mature dendritic spines [73]. mTORC1 activation stimulates protein and lipid synthesis promoting the increases of cellular mass, with expansion of plasma membrane, and axon guidance during development. The role of mTORC2 in brain development relies on the regulation of actin dynamics, facilitating the growth cone motility and path finding and elongation of neurites.
In adult brain, mTOR signaling evolved to control synaptic plasticity and processes underlying memory and learning [74]. Thus, in postmitotic neurons the mTOR pathway has significant influence on synaptic plasticity, neuronal polarity, neurotransmission, proteostasis, metabolic control, and stress responses including DNA repair [58]. Indeed, in adult CNS, mTOR is critical for long-term potentiation (LTP) in the hippocampus and, thereby, plays an important role in the process of learning and memory via protein synthesis-dependent consolidation of synapses [75]. The molecular mechanisms by which mTOR can regulate synaptic plasticity are very broad, and the analysis of RNAs regulated by the interaction of brain-derived neurotrophic factor (BDNF) with mTOR identified a number of proteins involved in synaptic plasticity, learning, and memory [59, 73, 75]. In addition, CamKIIα or PSD-95, regulating synaptic plasticity, also is dependent on mTOR activity [54, 76]. In aged brain, mTOR is profoundly involved in the regulation of protein homeostasis, through the on/off switch of translation and autophagy, to avoid the accumulation of toxic protein aggregates [58].
Recent reports focused on the role of mTOR in the development of neurodegenerative disorders, such as AD [61, 77, 78]. mTOR signaling is closely associated with the presence of two hallmarks of AD pathology: senile plaques, composed mainly of Aβ peptide, and neurofibrillary tangles (NFT), composed mostly of hyperphosphorylated tau protein. Studies from our and other laboratories showed an early hyperactivation of mTOR signaling, which is correlated with increased Aβ and tau hyperphosphorylation, in the brains of people with AD and DS [4, 79]. Interestingly, in DS the hyperactivation of mTOR is present in young individuals and is maintained in adulthood, in which AD pathology is marked. Thus we postulated that in people with AD and AD-like dementia, as in DS, the activation of mTOR signaling contributes to Aβ generation and the formation of NFT [77, 80] as shown in Fig. 1. Increasing evidence supports the notion that the activation of mTOR may contribute to senile plaque formation by regulating Aβ formation/clearance, which includes: (i) the regulation of APP expression; (ii) the inhibition of autophagy; and (iii) the interaction with signaling pathways such as PI3K/Akt, GSK-3β, AMPK, and insulin/IGF-1, involved in APP cleavage [80].

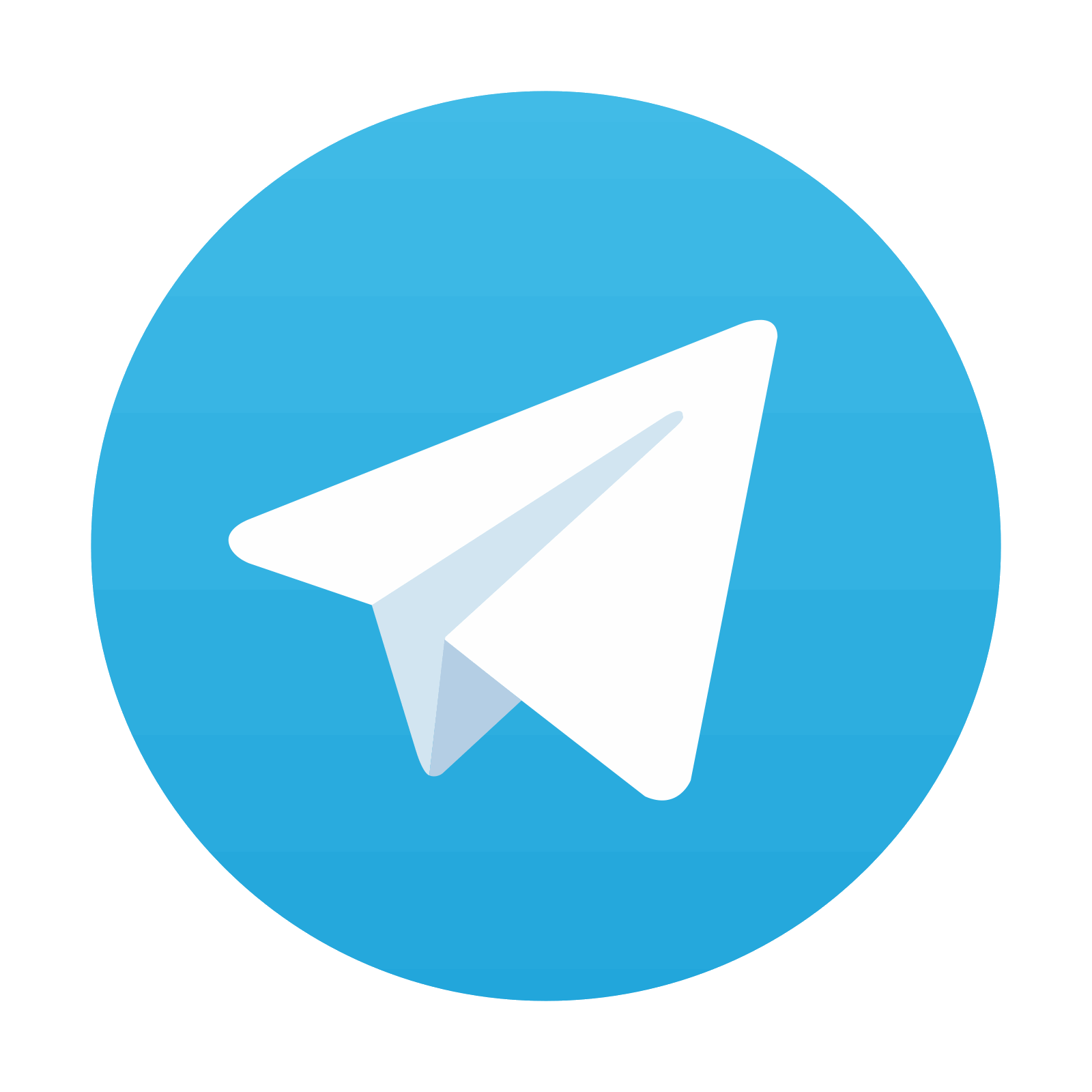
Stay updated, free articles. Join our Telegram channel
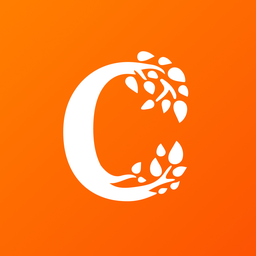
Full access? Get Clinical Tree
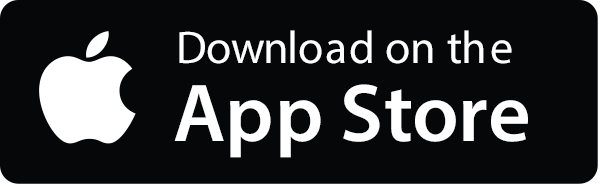
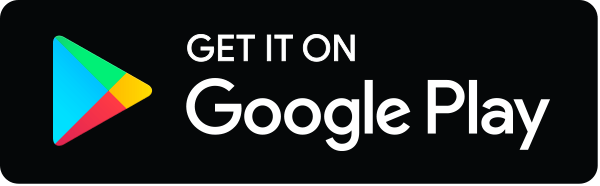