Chapter 48 The Neurobiology of Dreaming
Abstract
Cognitive Neuroscience and Dreaming
Dreaming is a universal human experience occurring during sleep in which fictive events follow one another in an organized, storylike manner and into which are woven hallucinatory, primarily visual, images that are largely congruent with an ongoing confabulated plot. Most often, this wholly imaginary experience is uncritically accepted in the same manner as are veridical waking percepts and events. The neuroscientific significance of dreams becomes apparent by considering just two of the many remarkable aspects of this universally human mental state. The first, dreaming’s “single-mindedness and isolation,” was eloquently described by Rechtschaffen,1 and refers to the dreamer’s absorption in the dream world and plot without awareness of an alternate reality in waking excepting rare “lucidity” (the awareness that one is dreaming). From this condition of limited insight, upon awakening, one abruptly regains sufficient insight to conceptualize alternate states of mind and decide which of these one is currently experiencing. The second is the occurrence in dreams of entirely de novo imagery, plots, personages, and even motor skills (e.g., flying), emotion (e.g., religious feelings) and memory (e.g., deja vu). Therefore, dreaming is an imprecise experiential simulacrum of waking resulting from neurobiological processes that must differ from those that generate waking consciousness. Because no published functional neuroimaging study has yet awoken subjects during dreaming to link subjectively experienced features of a dream report with immediately preceding brain activity, the wealth of information on the cognitive neuroscience of waking and the neurophysiology of sleep must be related to the less experimentally accessible dream state.
The Association of Dreaming With Behavioral State
Early speculation that rapid eye movement (REM) sleep was the exclusive physiological substrate of dreaming2 was soon followed by awakening studies showing substantial recall of mental experiences from non-REM (NREM) sleep.3 Nonetheless, REM reports are more frequent, longer, more bizarre, more visual, more motoric, and more emotional than are NREM reports (see review in Hobson et al.4). In an extensive review, Nielsen estimates an NREM mental-experience recall rate of 42.5% contrasting with 81.8% from REM and suggests that brain activation processes occurring outside polysomnographically scored REM (“covert REM”) may account for NREM dreaming.5
Recent Electrophysiological Findings
Fast and Slow Oscillations and Dreaming
REM sleep shows much more gamma frequency (30 to 80 Hz), fast brain waves (“oscillations” or “rhythms”) than does NREM sleep as measured by scalp electroencephalography (EEG),6,7 intracranial EEG (iEEG)8,9 and magnetoencephalography (MEG).6 In waking, these fast oscillations are associated with attention to stimuli and other forms of active or effortful cognition.10 During REM dreaming, fast oscillations have been hypothetically associated with cognitive and perceptual processing,11 memory processes12,13 and the temporal binding of dream imagery.14
In contrast to REM sleep, human slow-wave sleep shows very little gamma activity. It is instead associated with slower oscillations produced by recurrent interactions between the thalamus and cortex (intrinsic “corticothalamocortical” rhythms) such as sleep spindles and delta waves, and with the cortical slow (<1 Hz) oscillation that groups in time the other corticothalamocortical oscillations.15,16 The slow oscillation consists of periods of neuronal quiescence (hyperpolarized or “down” states) that alternate with shorter periods of rapid neuronal firing (depolarized or “up” states).17 Slow intrinsic oscillations may interfere with ongoing mental activity and lead to a lower frequency of dreams in NREM sleep.4
Cortical Connectivity in Sleep
Declines in phase synchrony (“coherence”) of EEG rhythms between different brain regions occurring during sleep relative to waking may reflect functional disconnections that contribute to the cognitive features of dreaming. Corsi-Cabrera and colleagues have shown that, compared to waking and NREM sleep, gamma frequency oscillations in REM sleep become desynchronized between areas near the front (anterior or frontal) and those near the back (posterior) of the brain.6,7,18 These investigators suggest that loss of anteroposterior gamma coherence reflects a functional disconnection of perception-related posterior cortical areas from executive control by the frontal cortex that may contribute to the “hypofrontal” (resembling frontal-lobe dysfunction) features and bizarreness of REM sleep dreaming.6,7,12,18 During REM sleep preceding dream reports, such frontoposterior gamma decoupling was accompanied by increased gamma coherence between posterior perceptual areas, possibly enhancing perceptual vividness in REM dreams.7 Similar sleep-related declines in gamma frequency coherence between corticocortical and corticohippocampal sites have been demonstrated using iEEG.12,13 Even EEG oscillations at frequencies slower than gamma have been shown to have lower coherence between anterior and posterior recording sites in REM versus NREM sleep7,19 despite the fact that slower oscillations tend to be more globally synchronized in NREM versus REM sleep20 due to the influence of intrinsic corticothalamocortical rhythms.16
Transcranial magnetic stimulation studies demonstrate reduced functional influence between different brain regions during REM sleep.21,22 Transcallosal inhibition of motor-evoked potentials is greatly reduced immediately following REM awakenings compared to both NREM stage 2 awakenings and waking,21 and transcranial magnetic stimulation of the premotor cortex during NREM sleep fails to persist or propagate to other sites as seen in waking.22
Dreaming and Phasic Activity in Sleep
In cats, a close temporal association exists between REM sleep rapid eye movements (REMs or REM saccades) and ascending potentials originating in the brainstem termed ponto-geniculo-occipital (PGO) waves.4,23 In the Activation-Synthesis (AS) hypothesis of dreaming,23 Hobson and McCarley suggest that the brainstem’s activation of the forebrain in REM allows the forebrain to synthesize dream scenarios based upon currently available information. They suggest that the PGO wave, originating in the pons and arriving at primary visual occipital cortex via the dedicated visual pathway through thalamic lateral geniculate nucleus (LGN), might be interpreted by the brain as visual information thereby leading to the visual hallucinosis of dreams.
Efforts to identify human correlates of the feline PGO wave have focused on the phasic periods of REM in which clusters of REMs and other transient muscle potentials occur. Phasic REM periods are contrasted with tonic periods that are lacking in or have only isolated REMs. Conduit and colleagues24 showed that in NREM sleep stage 2, awakenings preceded by eyelid movements (ELMs) yielded a higher frequency of visual imagery reports, and they suggested both ELMs and PGOs reflect an alerting mechanism similar to the startle response.
Early evidence of human PGO waves used scalp EEG recordings temporally locked to REMs (reviewed in Hobson et al.4 and Pace-Schott25). Compelling evidence for human PGO waves has recently emerged. First, using MEG tomography, Ioannides and colleagues26 have shown that correlated phasic activity in the pons and frontal eye fields begins before a REM saccade and intensifies with increasing temporal proximity to saccade onset, suggesting a buildup of neuronal excitability that culminates in the saccade. Because such activity is greatest in the pons, they suggest that accumulating excitability is driven by the pons with correlated frontal eye field activity representing feedback from pontine activity.26 Second, in a patient with Parkinson’s disease who was implanted with depth electrodes, Lim and colleagues27 described phasic signals, with wave form and temporal characteristics very similar to the feline PGO, originating in the pedunculopontine nucleus (PPT)—a structure at the pons-midbrain (mesopontine) junction crucial for generating the feline PGO (reviewed in Hobson et al.4).
Ogawa and colleagues28 provide event-related potential evidence that REMs generate perceptual experiences that could contribute to dream imagery. Brain potentials accompanying voluntary waking saccades were compared with those accompanying REM saccades. In waking, an EEG wave form known to reflect preparation for voluntary movement (“readiness potential”) was temporally locked to saccade onset, and another wave form believed to reflect visual processing of the saccade target was time-locked to saccade offset. In contrast, during REM, the wave form time-locked to saccade offset occurred in the absence of the presaccade readiness potential, suggesting that REM saccades themselves may trigger visual experiences. Using low resolution brain electromagnetic tomography, Abe and colleagues29 observed a pre–REM-saccade potential, temporally closer to saccade onset than waking readiness potentials, with current sources estimated to lie in anterior limbic regions (ventromedial, anterior cingulate, premotor, insular, temporopolar and parahippocampal cortices, basal forebrain, and uncus). Using MEG, Ioannides and colleagues26 also described REM-saccade onset linked current sources estimated to lie in the amygdala and orbitofrontal and parahippocampal cortices. The latter two groups link this presaccade limbic activity to phasically enhanced emotional processing.6,26,29
During phasic REM (versus tonic REM) sleep, increased gamma-frequency spectral power is observed across widespread scalp derivations using EEG6,11 and MEG6 as well as at specific orbitofrontal sites using iEEG.9 Gamma enhancement is accompanied by power attenuation at slower frequencies,9,11 and it is further enhanced during a 62.5 msec time window immediately preceding an actual REM saccade.6 Corsi-Cabrera and colleagues6 report that gamma coherence displays two distinct topographic changes during phasic and immediately presaccade REM relative to tonic REM. First, there is further uncoupling between frontal and parietal association areas that they suggest represents further decline in frontal control of posterior cortical perceptual-binding processes that may transiently increase cognitive distortions in dream mentation. Second, there is transient enhancement of frontolateral to midline gamma coherence that they suggest reflects phasically enhanced attentional processes subserved by midline structures.
In summary, EEG and MEG studies suggest that brain activity accompanying phasic REM sleep may be related to dream phenomena. Such phenomena include visual imagery,24,28 enhanced cognitive activity and attention,6,9,11 decoupling of executive control and perception,6,7,12,18 and enhanced emotional processing.6,26,29
Experimental Manipulation of Sleep Stage and Dreaming
Takeuchi and colleagues used a sleep interruption technique to elevate the frequency of sleep onset REM periods (SOREMPs) in normal subjects.30 Dreams elicited from SOREMPs were more bizarre and had different EEG correlates than NREM dreams that occurred at similar circadian phases preceded by similar sleep–wake durations.30 For example, whereas SOREMP dreams were correlated with the amount of REM in a sleep period and with absence of alpha frequency in the EEG spectrum, NREM dreams were correlated with the presence of arousals and the persistence of alpha rhythms.30
There is now evidence that “covert REM” processes can enhance NREM dreaming. For example, partial REM deprivation (REMD) increases both REM pressure and the dreamlike quality of NREM dreams at sleep-onset,31 a time when the EEG power spectrum is very similar to that during REM sleep.32 Additionally, when Suzuki and colleagues33 experimentally constrained sleep to 20 minute naps alternating with 40 minutes of waking across 3 days, NREM-only naps yielded more dream reports at times when, in REM-containing naps, REM duration was longest (peak at 8 AM). Therefore, increasing REM propensity by REMD or circadian manipulations can intensify NREM dreams.
Neural Activation Across the Wake-Nrem-Rem Cycle
Deactivation of frontal cortices is one of the first signs of human sleep observed using EEG, MEG, and functional neuroimaging (reviewed in Pace-Schott25 and Maquet34). Positron emission tomography (PET) studies of NREM sleep show declines in brain activity relative to waking both globally34 and in many specific regions of the subcortex and cortex35,36; findings now replicated using functional magnetic resonance imaging (fMRI).37 Global and regional cerebral activity further declines with the deepening of NREM sleep34,36–38 (although, on a microscopic time frame, activity in specific regions may become transiently elevated during the depolarized “up” phase of the slow oscillation39). Following sleep onset, EEG studies show greater slow wave spectral power in frontal versus posterior scalp-sites.40 Synchronization of slow-wave spectral power then spreads progressively to posterior regions,41 a trajectory also traveled by the slow (<1 Hz) oscillation.42
From these deactivated NREM conditions, in REM, there is then a prominent increase of neural activity in subcortical brain regions, including the pons and midbrain,35,43 thalamus,35,43 basal ganglia,35 limbic subcortex including the amygdala,43 hypothalamus, and ventral striatum.35 Increases are also seen in limbic-related cortices anteriorly in the rostral and subcallosal anterior cingulate,35,43 the anterior insular, more posterior (caudal) orbitofrontal and paracingulate Brodmann area (BA) 32 cortices, BA 10 in medial prefrontal cortex (mPFC),35 as well as more caudally in the parahippocampal gyrus and temporal pole.35 Certain visual association cortices (areas that process higher-order aspects of vision) are also active.35,44 However, multiple neuroimaging modalities show that lateral prefrontal cortices remain deactivated after the transition from NREM to REM sleep.35,43–45
When REM sleep is directly compared to waking, there is relative deactivation of the lateral prefrontal cortex.35,43,45 Maquet and colleagues45 show that regions most consistently hypoactive in REM compared to waking include middle and inferior frontal gyri as well as inferior parietal and temporoparietal junction association cortices. However, compared to waking there is greater activation of limbic and paralimbic regions.35,43,45–47 Nofzinger and colleagues46,47 have termed this area the “anterior paralimbic REM activation area” (APRA) and describe it as a “bilateral confluent paramedian zone which extends from the septal area into ventral striatum, infralimbic, prelimbic, orbitofrontal and anterior cingulate cortex”p. 192 including the hypothalamus, ventral pallidum, hippocampus, and uncus, as well as supplementary motor, pre- and subgenual anterior cingulate and insular cortices.46
Although lacking the temporal resolution of EEG and MEG, glucose metabolic 18FDG (2-deoxy-2[18F] fluorodeoxyglucose) PET studies correlating REM density with regional glucose metabolism also suggest activation of arousal, attention, and emotion networks in association with REM saccades.48 Using fMRI, Hong and colleagues49 have recently reported widespread blood oxygen level–dependent activity correlated with REMs that includes not only brainstem, thalamic, and limbic areas, but also primary and secondary sensory cortices and areas subserving oculomotor control and visuospatial attention. Recent oxygen use (H215O) PET50 and fMRI51,51a studies also correlate REMs with activation of structures corresponding to the feline PGO wave including the rostral brainstem,50,51a LGN, and occipital cortex.50,51,51a Phasic REM episodes arising from a tonic REM background show characteristic fMRI blood oxygen level–dependent changes in forebrain activity that include increased functional connectivity (the tendency to activate together) between the thalamus and a broad cortical-limbic-striatal network.52 Wehrle and colleagues52 suggest these changes represent activation, during phasic REM, of networks important to memory and emotional processing. Therefore, like EEG and MEG studies, functional neuroimaging suggests that phasic REM is associated with brain activity that might reflect intensified dream imagery, attention, and emotion.
Dreaming and “Default Mode” Networks
Regions that characteristically show deactivation in response to a wide variety of goal-directed cognitive tasks have been termed the brain’s default mode.53,54 In the absence of an exteroceptive focus, these regions are believed to carry out cognitive and emotional processes relating to self-directed concerns (“self-referential cognition”).53,54 Default network regions include a posteromedial parietal region (posterior cingulate, precuneus, and retrosplenial cortices), and a lateral-inferior-parietal/superior-temporal region, the hippocampal formation (hippocampus and parahippocampal cortex), ventromedial prefrontal cortex (vmPFC), and dorsomedial prefrontal cortex (dmPFC).53,54 Posterior default-network regions are linked to autobiographical memory retrieval and anterior regions with self-referential cognition.53,55 Default network activity in the vmPFC has been associated with the integration of internal stimuli reflecting bodily sensations with external, sensory stimuli54 as well as a feelings-based appraisal of whether or not such stimuli are self-related.55 Activity in the dmPFC has been associated with self-referential cognitive, behavioral, and emotional imagination (“simulation”) and its rehearsal,53,54 as well as cognitive appraisal of self-related stimuli.55 The dmPFC regions are also strongly activated by social cognition tasks such a those that engage abilities to infer others’ feelings, thoughts, and intentions (“theory-of-mind”).55
The default network contains two interacting subsystems, centered on the dmPFC and hippocampal formation respectively, activity in both of which correlates positively with a core network (vmPFC, posterior cingulate, retrosplenial and inferior parietal cortices) but negatively with each other.53 The hippocampal formation may access personally relevant memory whereas the dmPFC may simulate future scenarios.53 Notably, both retrospective (autobiographical) remembering and prospective simulation (imagining the future) engage much the same regions of the default network.53
Using fMRI, temporal synchrony of low frequency (0.01 to 0.1 Hz) spontaneous fluctuations of the blood oxygen level–dependent signal in waking reflects anatomical and functional connectivity among regions of the default network.56 Such synchronized fluctuations persist into attentional lapses following sleep deprivation,57 light NREM sleep58 and even stage 2 NREM sleep,59,59a but not slow-wave sleep59,59a (although default areas may transiently activate during the “up” phase of the slow oscillation39). However, reactivation of default network structures during REM, as measured by PET, is only partial.46,47 The anterior default network, vmPFC, and portions of dmPFC, as well as hippocampal and parahippocampal areas, reactivate.35,43,44,46,47 In contrast, posterior, parietal default network areas, especially the lateral inferior parietal and posterior cingulate cortex, remain deactivated.35,43,45
REM sleep dreams may, therefore, reflect default network processing with disconnection between network subsystems due to a partially deactivated core. Specifically, self-referential, emotional and social cognitive simulation, subserved by anterior default-network structures (vmPFC, dmPFC), may be isolated from hippocampally-retrieved autobiographical memory due to absence of memory-access facilitation by posterior default network structures (posterior cingulate and retrosplenial cortices). With limited access to episodic, autobiographical memory, REM-sleep dreaming might simulate lifelike events53 in a manner unconstrained by past realities.60
The Neurochemistry of Dreaming
Three major neurochemical hypotheses explain differences between dreaming and waking consciousness. The activation-synthesis and activation-input-modulation models of Hobson and colleagues suggest that the massive increase in cholinergic (relative to noradrenergic and serotonergic) activation from the ascending reticular activating system (ARAS) during REM sleep contributes strongly to the unique nature of dream consciousness.4,23 Solms suggests that stimulation of limbic and prefrontal reward networks by dopaminergic projections from the midbrain ventral tegmental area (VTA) generates motivational impulses that initiate dreaming.61 Gottesmann suggests that dopaminergic stimulation of the cortex during REM, in the absence of waking’s inhibitory serotonergic and noradrenergic modulation, allows emergence of psychotomimetic (psychosis-like) aspects of dream consciousness.62
Acetylcholine
The activation-synthesis23 and activation-input-modulation4 models suggest that forebrain activation in REM dreaming originates in ascending activation of the thalamus by mesopontine cholinergic nuclei. Much evidence exists for cholinergic enhancement of both REM and dreaming. Higher mesopontine-brainstem derived acetylcholine (ACh) concentrations during wake and REM sleep versus NREM sleep are seen in the thalamus, including the LGN.63 Cholinergic stimulation potentiates REM sleep when microinjected into the animal brainstem or when systemically administered to humans.4 Cholinesterase inhibitors can induce REM sleep with dreaming,64 and increase nightmares65 and hypnagogic hallucinations.66 Transdermal nicotine67 and its partial agonist varenicline68 intensify dreams. Nightmares induced by beta-blockers69 probably result from disinhibition of cholinergic-brainstem REM-generating mechanisms.70
Nonetheless, the most common pharmacological precipitant of waking hallucinosis is the delirium resulting from blockade of muscarinic ACh receptors.71 Moreover, extensive cholinergic deficits may cause the visual hallucinations of Lewy body dementia.71 The apparently paradoxical association of waking hallucinosis with hypocholinergic and REM dreaming with hypercholinergic brain conditions may arise because dreaming and waking hallucinations are differently generated. Perry and Perry71 suggest that, in waking, ACh from the basal forebrain excites the GABAergic interneurons of the cortex thereby inhibiting cortical pyramidal neurons and improving the signal-to-noise ratio at their synapses. In contrast, during REM sleep, ACh from the mesopontine brainstem inhibits GABAergic interneurons of the thalamus thereby disinhibiting, as well as directly exciting, thalamocortical neurons (thalamic neurons that project to and excite the cortex) possibly, in part, via nicotinic receptors that are especially abundant in the thalamus.71 Therefore, reduced ACh in waking allows intrusion of weaker inputs, whereas increased ACh in REM sleep excites the cortex in the absence of any perceptual input—two differing conditions but both conducive to hallucinosis.71 Notably, by combining a cholinergic challenge with fMRI, Furey and colleagues72 have shown that, in waking, ACh enhances the activity of visual association cortices (in REM sleep, possibly favoring hallucination) while at the same time reducing dorsal prefrontal cortical activity (in REM sleep, possibly diminishing logic and insight).
Dopamine
Because mean firing rates of dopaminergic cells in the substantia nigra pars compacta and VTA do not vary with behavioral state in the same manner as do the other aminergic nuclei, dopamine (DA) has received less study by sleep and dream researchers (reviewed in Hobson, Pace-Schott, and Stickgold4 and Hobson and Pace-Schott70). However, a key role is assigned to DA in reward-based61 and psychotomimetic62 theories of dreaming. Recent findings in rodents have begun to identify roles for DA in REM sleep. For example, enhancing REM intensity by prior REMD increases c-fos expression in the VTA,73 and DA concentrations in the mPFC and nucleus accumbens are greater during REM sleep than they are during NREM sleep.74 Because transition to a burst-firing mode releases more presynaptic DA,74 synaptic DA concentrations can vary without change in dopaminergic neurons’ mean firing rate. Dahan and colleagues75 have demonstrated just such a transition during REM sleep in DA neurons of the VTA. The REM-related increase of burst firing in the VTA may result from increased cholinergic excitation from the PPT74 that innervates76 and strongly excites77 the VTA. Although L-dopa and certain other dopaminergic agents can enhance dreaming in persons with Parkinson’s disease and other clinical conditions (reviewed in Solms,61 Pagel,69 and Hobson and Pace-Schott70), psychostimulants are not associated with dream enhancement, neuroleptics do not prevent dreaming, and there exist DA agonists that reduce, and antagonists that enhance, dreaming.70 Thus DA’s dream effects may be dependent on dosage, as well as receptor type and location.
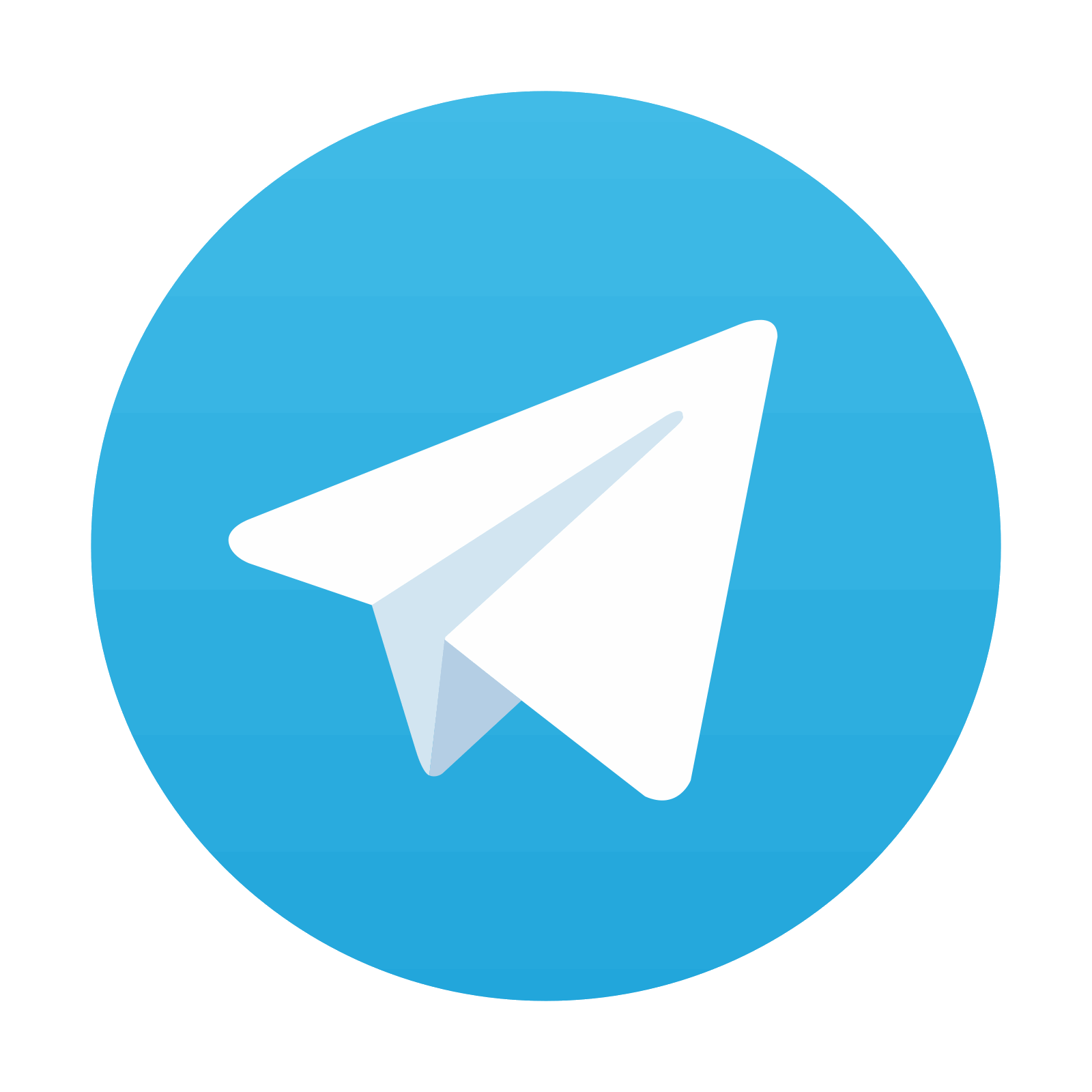
Stay updated, free articles. Join our Telegram channel
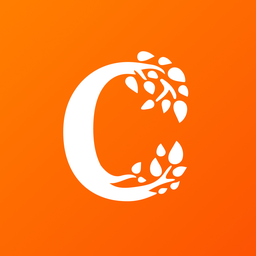
Full access? Get Clinical Tree
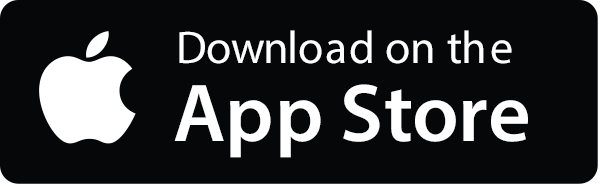
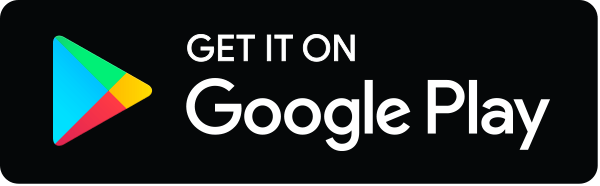