Amygdala: functional subdivisions and basic organization of flow of information. The amygdala is not a unitary structure, and consideration of its anatomical subdivisions and circuitry is essential. (A) One of the best characterized models of information flow and intra-amygdala circuitry involves the basolateral nuclear complex (which subsumes lateral and basal nuclei) and the central nuclear complex (which consists of a lateral [light red] and medial component [dark red]); these two complexes are considered the primary input and output components of this circuit, respectively. The basolateral complex receives cortical and thalamic sensory input. The lateral nucleus projects to the basal and central nuclei (lateral component); the basal nucleus projects to the central nucleus (medial component), as well as the intercalated masses (IM). The IMs are clusters of GABAergic inhibitory neurons, nestled between basolateral and central nuclei, which play a critical role in regulating central nucleus activity. The central nucleus projects to various subcortical regions, such as the hypothalamus, bed nucleus of the stria terminalis (BNST), nucleus basalis (major site of acetylcholine [ACh] ascending projection neurons), and various brainstem regions that regulate neurotransmitter systems, autonomic function, innate psychomotor and visceromotor responses, and descending pain-regulation pathways. Central nucleus efferents are believed to consist of tonically active inhibitory projection neurons that activate their downstream targets when their activity is inhibited [30,31]. (B)–(C) Studies of the amygdala in humans have typically considered it as a single functional unit, mainly due to its amorphous shape and relatively small size. Recent imaging studies have demonstrated the ability to differentiate the human amygdala in vivo into the three main nuclear complexes: basolateral, central (centromedial), and superficial (cortical) [32].
Owing to its relatively small size and the amorphous organization of its functional subdivisions, the amygdala has typically been assessed as a unitary structure in human imaging studies. Only until recently have the functional subdivisions of the amygdala been neuroanatomically delineated in human imaging studies (Figures 13.1B and 13.1C) [32]. As will be highlighted throughout this section, taking into account the functional subdivisions of the amygdala is critical to better resolve the relationship between amygdala structure/function and aggression.
A relatively consistent finding of structural imaging findings has been an association between aggression and reduced amygdala volumes. In a study of healthy, nonclinical female participants (N = 20) with levels of trait aggression not outside the normal range, Matthies et al. [33] found that both right and left whole amygdala volumes were smaller in those who scored higher than the cohort median on a commonly used measure of trait aggression (Lifetime History of Aggression; LHA) relative to those with LHA scores below the cohort median.
The volumes of both the right and left amygdala were also negatively correlated with LHA scores among all participants combined. Finally, specificity of this effect for aggression was supported by the absence of correlations between amygdala size and other clinical measures, namely general psychopathology, trait anxiety, and state depression. An important caveat of these findings, however, is their applicability to clinical populations, pathological forms of aggression, and males.
Pardini et al. [34] examined amygdala volumes in men (N = 56) who had been psychometrically assessed at three time points: (1) childhood and adolescence; (2) at the time of an MRI at 26 years of age; and (3) prospectively, approximately three years after the MRI. Notably, these participants manifested a broader range of aggression and psychopathology than in the Matthies et al. study, described above. Similar to what Matthies and colleagues observed, however, both the left and right whole amygdala volumes were negatively correlated with a number of measures of trait aggression performed at the time of MRI in adulthood. Remarkably, aggression that had been assessed in childhood and adolescence was also negatively related to adulthood amygdala volumes. Finally, both left and right whole amygdala volumes were found to be smaller in men who engaged in violent acts within the 3-year post-scan follow-up interval, even after adjusting for ratings of aggression prior to the post-scan assessment. Relations between amygdala volumes and psychopathy, a construct commonly associated with aggression, were somewhat mixed, however. Psychopathy assessments performed contemporaneously with the structural MRI in adulthood were not related to amygdala volumes; however, significant associations did exist between adulthood amygdala volumes and psychopathic features assessed in childhood/adolescence and at the 3-year post-scan follow-up assessment. Taking into account the different forms of aggression (namely reactive and proactive – which have different relationships with psychopathy) and characterizing amygdala subregions in future studies may allow for these complex relationships to be better resolved.
In order to enhance the specificity of the relationship between amygdala structure and aggression, studies have begun to take into account the anatomical functional subdivisions of the amygdala (described earlier, and depicted in Figures 13.1B and 13.1C). Gopal et al. [35] parcellated the amygdala into ventral and dorsal portions (roughly approximating the basolateral and centromedial nuclear complexes, respectively) and examined the relationship between amygdala volume and trait aggression in a mixed population of psychiatric patients with a wide range of diagnoses (N = 41). Unlike the two studies cited above, a relationship between whole amygdala volumes and trait aggression (as assessed by the LHA) was not observed. However, planned comparisons revealed that the volume of the left dorsal amygdala, specifically, was negatively correlated with the LHA. In contrast, they found that the closely related trait, (motor) impulsivity, was positively correlated with both the right and left ventral amygdalae, but not with either dorsal subdivisions. These findings highlight the importance of taking into consideration the anatomical and hemispheric specialization of the amygdala, as well as the potential confounding role of closely related traits that may in fact have contrasting neuroanatomical correlates.
Finally, Bobes et al. [36] reported that in a community sample of adult men (N = 54) whom were classified as either aggressive (N = 25) or non-aggressive (N = 29) based on a self-report measure of reactive aggression, both right and left whole amygdalae volumes were found to be smaller in the aggressive compared to the non-aggressive group. They also found that in the aggressive compared to the non-aggressive group, volumes of both the dorsal and ventral portions of the right amygdala were decreased, however, only the dorsal component of the left amygdala was reduced. Furthermore, while volumes of the left and right amygdala were negatively correlated with a general measure of trait aggression (i.e., not one specifically designed to differentiate proactive from reactive aggression), only the left amygdala correlation remained significant after correcting for multiple comparisons. Volumes of neither the left nor right amygdalae were correlated with measures of the interpersonal/affective dimensions of psychopathy. Curiously, an unexpected finding was that right amygdala volumes were found to be negatively correlated with a measure of proactive aggression.
Therefore, in a fairly wide range of populations, an inverse association between amygdala volume and trait aggression appears to be a relatively consistent finding. Further, there is growing evidence that taking the functional subdivisions of the amygdala into account is necessary in order to better resolve the amygdala–aggression relationship. Preliminarily, it appears the left, dorsal amygdala may have a distinct, albeit not exclusive, role. Preferential involvement of the dorsal amygdala points to altered amygdala output functionality; however, it will be necessary to determine the precise nature of this functional effect. Further, involvement of the left amygdala invokes a role for explicit/ conscious and effortful mechanisms of affect processing, as opposed to implicit/subconscious and automatic processes, which have been associated with the right amygdala [37].
Thus far, we have focused on the relationship between amygdala structure and trait aggression. The functional significance of these structural changes, however, remains unclear. For example, is reduced amygdala volume associated with amygdala hypoactivity or hyperactivity? Thus, we now turn to functional studies that examine the amygdala’s involvement in aggression.
We recently examined patterns of regional brain metabolic activity in patients comorbid for IED and BPD (N = 38) in comparison to healthy control participants (N = 36) using [18]fluoro-deoxyglucose positron emission tomography (FDG-PET) in conjunction with a laboratory aggression task, the Point Subtraction Aggression Paradigm (PSAP) [38]. Importantly, FDG-PET was performed twice, under two different PSAP settings: a provoked condition, in which a fictitious competitor would “steal” or subtract points from the participant, and an unprovoked condition, for comparison. As expected, the BPD-IED group exhibited more aggressive behavior during the baseline (unprovoked) condition than the control group; and under the provoked condition, both groups exhibited an increased frequency of aggressive responding toward the (fictitious) competitor relative to the unprovoked state, although the level of aggressive behavior in the BPD-IED group was still greater than that of controls during provocation.
In terms of amygdala metabolic activity, we observed group differences in baseline amygdala activity, as well as in response to provocation: in the unprovoked state, amygdala metabolic activity was lower in the BPD-IED group compared to controls; and in response to provocation, amygdala activity increased in the BPD-IED group and decreased, albeit slightly, in the control group, despite the fact that both groups exhibited a similar degree of increased aggressive responding in the provoked compared to unprovoked condition [38]. Although there are important caveats to interpreting these findings, such as not accounting for amygdala functional subdivisions, they do suggest that amygdala activity is more labile, and perhaps less well modulated, in those with pathological forms of aggression.
A role for enhanced amygdala responsivity in aggression has also been demonstrated in studies employing functional magnetic resonance imaging (fMRI). For example, Coccaro et al. [39] previously described enhanced amygdala activity in response to viewing threatening facial expressions (specifically, anger and fear) in aggressive individuals relative to controls. Investigators, therefore, have taken advantage of this paradigm as a means to interrogate the neural structures and circuitry underlying aggression, without having to elicit aggressive behavior in the laboratory setting.
In addition to their analysis of amygdala volume, the study by Bobes et al. [36] discussed above, also employed fMRI to examine amygdala activation in response to fearful facial expressions. More specifically, they assessed the difference between amygdala responses to fearful and neutral faces (F/N-difference), with the neutral facing serving as a type of control stimulus. They found that in aggressive compared to non-aggressive participants, the F/N-difference was reduced, specifically, in the left dorsal amygdala. Moreover, among all participants, the amygdala F/N-difference was significantly negatively correlated with factors related to impulsive aggression, but not with the interpersonal/ affective dimensions of psychopathy.
Such a reduction in the F/N-difference potentially signifies that amygdala responses to fearful faces are reduced in aggressive individuals, which would conflict with the notion of amygdala hyperactivity in aggression. However, Bobes et al. [36] found that amygdala responses to both fearful and neutral faces were in fact increased in the aggressive group relative to the non-aggressive participants; however, the increase for neutral faces was greater than that of fearful faces. Thus, it was the increase in amygdala response to neutral faces that primarily determined the reduced F/N-difference. In other words, it is not that the aggressive group demonstrated less amygdala activity in response to fearful faces compared to the non-aggressive group, but rather, they exhibited greater amygdala activation to both fearful and neutral faces compared to the non-aggressive group. However, the neutral face-difference was even larger than that associated with fearful faces. Thus, the relatively lower F/N-difference in the aggressive group should not be equated with amygdala hypoactivity in response to socially threatening stimuli, but rather, amygdala hyperactivity in response to both fearful and neutral faces, but to a greater extent with respect to the neutral stimuli.
The last functional study we will discuss was performed Lozier et al. [40] in which they characterized the role of the right amygdala in proactive aggression by examining differences in the processing of fearful facial expression in adolescents with conduct problems (N = 30) compared to a healthy adolescent group (N = 16). It should be noted that this study differs from the others discussed above in that it consists of an adolescent as opposed to adult population, and is specifically focused on the right amygdala and proactive aggression.
Initially, Lozier et al. unexpectedly found an apparent absence of differential right amygdala activity to fearful faces in adolescents with conduct problems compared to controls; however, this was found to be due to “statistical suppressor effects” of associated pathological personality dimensions that exhibited contrasting neural correlates [40]. Specifically, they found that callous-unemotional (CU) traits (a component of psychopathy) and externalizing behaviors were negatively and positively correlated, respectively, with right amygdala responses to fearful faces. Thus, even though CU traits and externalizing behaviors commonly co-occur, they nevertheless exhibited a contradistinctive relationship with respect to right amygdala response to fearful faces. Such contrasting effects between highly covariant phenomena are a potential source of heterogeneity and inconsistency between studies.
Next, Lozier et al. found that, similar to CU traits, proactive aggression was also negatively correlated with amygdala response to fearful faces, while covarying for externalizing traits [40]. Further, they described that right amygdala hypoactivity to fearful faces mediated the relationship between CU traits and proactive aggression.
In other words, altered right amygdala functionality may be necessary for CU traits to manifest in terms of proactive aggression. The role of the right amygdala’s differential response to fearful faces serving as link between CU traits and proactive aggression was shown to be specific in three ways. First, right amygdala responses to angry or neutral faces did not mediate a link between CU traits and proactive aggression. Second, right amygdala responses to fearful faces did not link CU traits and reactive aggression. And last, right amygdala responses to fearful faces did not link externalizing behaviors and reactive aggression.
Prefrontal cortex and prefrontal–amygdala interactions
Studies of aggression have primarily implicated the limbic PFC, which includes portions of the orbitofrontal cortex (OFC) and the anterior cingulate cortex (ACC). Generally speaking, the OFC and ACC are both involved in the integration of affective, sensory, and cognitive processes, for the purpose of generating state representations that are coherent, nuanced, and textured, as well as behavioral, cognitive, and affective responses that are modulated, contextually appropriate, and flexible [41,42]. The OFC is considered to play more of a sensory or perceptual role, e.g., appraising the motivational value and affective valence of stimuli; the ACC, on the other hand, is more closely associated with determining “actions” or “responses.”
Surprisingly, only a handful of studies have examined structural differences of the OFC and ACC in aggression. For example, smaller left OFC gray matter volume has been shown to be related to greater levels of trait aggression [43]. Similarly, greater right/left OFC volume ratios were also associated with greater trait aggression in those with a history of affective illness [44]. In child and adolescent populations, reduced right ACC volumes have been associated with increased aggression [45,46].
Early, functional studies indicated an association between aggression and reduced ventromedial PFC basal metabolic activity [47] and attenuated metabolic activation to serotonergic agents [48]. A recent study of ours, however, revealed a more complex picture. While metabolic activity of the OFC was lower under control conditions in a BPD-IED group compared to healthy controls, with provocation and increased aggressive responding, the OFC exhibited a relative increase in metabolic activity in the BPD-IED group, whereas in the healthy control group, OFC activity was reduced with provocation and increased aggressive responding [38]. The specificity of this effect for the OFC was demonstrated, as other prefrontal regions (medial, anterior, dorsolateral) did not differ between groups. Finally, we found that the correlation between medial OFC activity and aggressive responding, observed in healthy controls, was absent in the BPD-IED group. These findings suggest that the role of the OFC in the pathophysiology of aggression may not be best ascribed to a “hypoactivity” mechanism, but rather a “disconnection” one. As we will describe below, it has been useful to characterize the neural basis of aggression in terms of differential connectivity or coupling between the ventromedial PFC and the amygdala.
Coccaro et al. [39] described that while viewing angry faces, IED patients (N = 10) exhibited increased amygdala and decreased medial OFC activity compared to control participants (N = 10). Furthermore, it was described that among controls, amygdala activity was negatively correlated with that of the medial OFC (denoting the two regions were functionally coupled), whereas in the aggressive group, no amygdala–OFC coupling was observed [39].
We will first describe here (and in Figure 13.2) an overview of the basic neurobiology of amygdala–PFC connectivity before addressing its specific role in aggression. Work in primates has revealed a number of general principles regarding the structural basis of amygdala–PFC interconnectivity. First, the limbic portions of the PFC, namely, the OFC and ACC, are the prefrontal regions that exhibit the most robust reciprocal anatomical connections with the amygdala – particularly, the posterior portion of the OFC [49,50] and the pregenual and subgenual (i.e., caudal) ACC (Figure 13.2A) [49]. Amygdala innervation is more scant and unidirectional in the more dorsal and lateral portions of the PFC [49]. Second, as illustrated in Figure 13.2A, the ACC sends more projections to the amygdala than it receives, whereas the posterior OFC receives more amygdala projections than it sends. This is consistent with the more “action”- or “response”-oriented functions of the ACC, and the “sensory” or “perceptual” role of the OFC. Finally, the termination patterns of OFC and ACC projections to the amygdala differ (see Figures 13.2C and 13.2D for details), which suggests that the former is an arbiter of central nucleus activity, whereas the latter modulates how the basal nucleus processes sensory input [51].
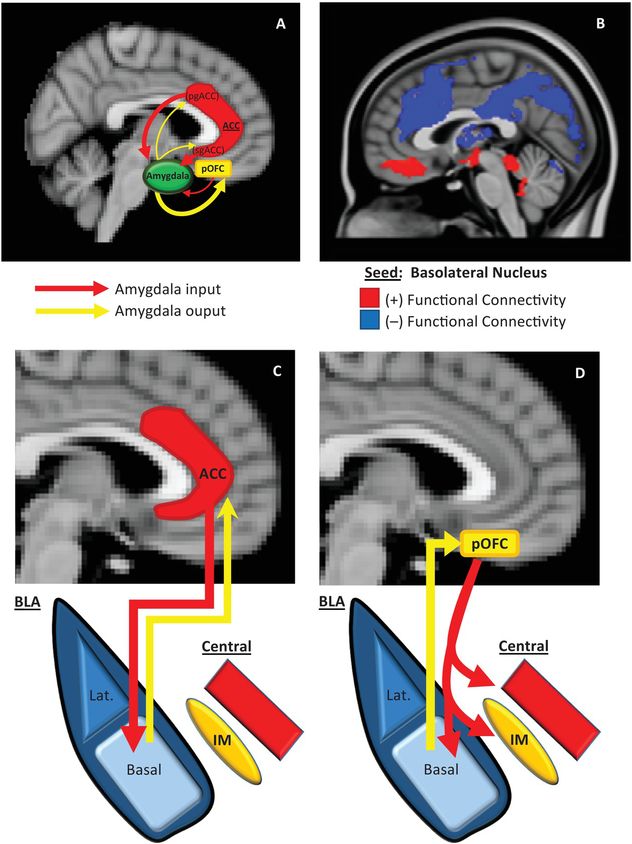
Prefrontal–amygdala circuitry. Altered prefrontal amygdala circuitry has been implicated in the neurobiology of aggression. (A) Preclinical studies in primates and rodents have demonstrated that the portions of the prefrontal cortex most robustly interconnected, anatomically, with the amygdala include two basic regions: the posterior extent of the orbitofrontal cortex (pOFC) and the caudal portions of the anterior cingulate cortex (ACC), roughly corresponding to the subgenual (sg)ACC and pregenual (pg)ACC. Anatomical connectivity between the amygdala and PFC, in general, decreases as it moves toward the dorsal and lateral aspects of the PFC (not depicted in this figure). Although there is significant bilateral anatomical connectivity between the amygdala and both the ACC and pOFC, studies in primates have also demonstrated relatively greater ACC-to-amygdala projections than amygdala-to-ACC projections; the pOFC, on the other hand, receives a greater number of projections from the amygdala compared to projections the pOFC sends to the amygdala. This has been considered to signify that the ACC is more of a sender of amygdala–PFC projections, whereas, the pOFC is more of a receiver [49,50]. (B) These structural data in primates are broadly consistent with intrinsic functional connectivity studies of the amygdala complex in humans, although the latter provides, of course, relatively less anatomical resolution. As illustrated in (B), the subgenual/orbital portion of the medial PFC exhibits positive functional connectivity (red) with the basolateral amygdala complex, which suggests a predominantly feed-forward relationship. The pregenual medial PFC, on the other hand, exhibits negative functional connectivity with the basolateral amygdala complex (blue), which indicates a feed-back role [52]. There is a general absence of functional connectivity with the more dorsal and lateral (not shown) PFC, which is similar to primate structural findings. (C)–(D) There are important anatomical differences in the termination patterns of projections from the OFC and ACC to the amygdala in the primate [51]. Projection neurons from the ACC terminate broadly throughout the basal nucleus (as well as other amygdala nuclei), whereas OFC efferents are relatively unique in that they terminate among the intercalated masses or IMs (clusters of GABAergic inhibitory neurons that regulate central nucleus activity), as well as the central nucleus itself. Therefore, the pOFC may act as a direct arbiter of central nucleus activity, and therefore, determine the extent to which a stimulus representation is infused with a visceral/autonomic or “somatic” component. The ACC, on the other hand, may influence more broadly how sensory inputs to the amygdala are processed. It remains unclear how pregenual and subgenual portions of the ACC may differ in this respect, however.
Human studies of amygdala–PFC connectivity, while unable to provide the same level of neuroanatomical resolution as animal studies, have been broadly consistent with the preclinical findings described above, and have offered additional functional information. As shown in Figure 13.2B, “resting state” or intrinsic functional connectivity studies have demonstrated that the basolateral nuclear complex is strongly (negatively) coupled with the ventral, pregenual PFC and (positively) coupled with the subgenual/orbital PFC. Also, there is an absence of connectivity between the basolateral complex and the dorsal and lateral portions of the PFC [52]. Notably, the negative connectivity between the ventral pregenual PFC and basolateral amygdala is consistent with a primarily “top-down,” inhibitory feed-back process, whereas the positive connectivity with the subgenual/orbital PFC suggests a possible “bottom-up,” feed-forward process.
We will now review specific studies in which aggression has been examined in terms of differential amygdala–PFC connectivity. As described earlier, Coccaro et al. [39] found that the coupling between the amygdala and medial OFC, which occurred while healthy participants viewed angry faces, was absent in patients with IED. Similarly, in a more recent study using restingstate fMRI in patients with schizophrenia (N = 25), Hoptman et al. [53] demonstrated that functional connectivity between the amygdala and ventral PFC was significantly decreased compared to healthy controls (N = 21), and critically, they found a significant negative correlation between trait measures of aggression and amygdalo-frontal connectivity. Similarly, studying a nonclinical male population (N = 16), Fulwiler et al. [54] observed that trait anger was inversely correlated with functional connectivity between the amygdala and contralateral medial OFC – most strongly between the right amygdala and left medial OFC. Furthermore, they found that a measure of anger control, i.e., the propensity to try to suppress one’s response to angry feelings, was positively correlated with amygdala–OFC functional connectivity. An important question for future studies is to what extent altered amygdala–PFC connectivity is structural or neuromodulatory. In a recent study of a large cohort of healthy male participants (N = 93), Beyer et al. [55] did not observe a significant difference in a measure of structural connectivity between the amygdala and PFC in those with high compared to low trait-aggressiveness. It will be essential to extend such studies to clinical/pathological manifestations of aggression, however.
In terms of a neuromodulatory basis for altered amygdala–PFC connectivity, the serotonergic system is emerging as a likely candidate [56]. For example, pharmacological antagonism of 5-HT2A receptors enhances medial OFC–amygdala connectivity during the processing of fearful faces [57]. As will be discussed further below (in Receptor subtypes: 5-HT2A), this is consistent with our finding that state aggression in personality disordered patients with IED is positively correlated with 5-HT2A receptor availability in the OFC [58]. Dietary tryptophan depletion, which acutely lowers central serotonin synthesis, disrupts amygdala connectivity with the ventral ACC and ventrolateral prefrontal cortex, specifically when viewing angry faces [59]. Finally, polymorphisms of the 5-HT transporter have been associated with differential amygdala–PFC connectivity [60,61].
Therefore, it is becoming increasingly clear that while both the amygdala and ventral and medial aspects of the PFC are involved in aggression, it is essential that their concerted function be examined. Further, it is critical that the neuroanatomical specificity of amygdala–PFC interactions be taken into account, as connectivity with the amygdala differs not only between the ACC and OFC, but within functionally distinct subregions of each of these structures.
Striatum
The striatum integrates widespread, direct cortical input, and projects to pallidal structures that ultimately, via direct and indirect pathways, modulate thalamocortical activity. Through its involvement in this circuitry, the striatum plays a critical role in the appropriate selection and inhibition of various competing, motor, cognitive, and emotional response sequences [62].
The ventral and dorsomedial portions of the striatum are notable candidates for involvement in aggression, given their role in “goal-directed,” “motivated,” and “value-based” processes. The ventral striatum is believed to be involved in processing the anticipated value of “outcomes” or “events,” whereas the dorsomedial striatum underlies the expected value of “actions.”
Therefore, the ventral striatum conceivably may determine, for example, one’s expectations from social interactions, and therefore contribute to phenomena, such as excessive frustration from interpersonal slights, that are common precipitants of impulsive aggression. The dorsomedial striatum, on the other hand, may be involved in determining the value of certain responses to frustration, such as assaultiveness as opposed to composed restraint.
Furthermore, striatal parameters that determine event and action values are modulated by the dopaminergic and serotonergic systems. Dopamine is believed to encode the expected value of an event or action and also the degree of increase or decrease in synaptic dopamine after an event reflects the extent to which an outcome exceeded or fell short of expectations, respectively. Through its modulation of striatal dopamine, the serotonergic system determines the temporal discount parameter, i.e., how much the delay of receiving a reward diminishes its value.
Buckholtz et al. [212] found that in a community sample of adults without a substance abuse history, the impulsive-antisocial dimension of psychopathy was positively correlated with both presynaptic dopamine release-capacity in the ventral striatum, and ventral striatal activity while waiting for a monetary reward. These findings suggest that immoderate ventral striatal function may be associated with impulsive aggression (a component of impulsive antisociality) by leaving an individual susceptible to disproportionate frustration, such as undue sensitivity to interpersonal slights and social rejection, which are common precipitants for aggression.
The dorsomedial striatum, in concert with the serotonergic system, has also been implicated in aggression. Crockett et al. [63] performed fMRI while participants engaged in a social trust paradigm in which they could accept or reject, and found that dorsomedial striatal activity was directly correlated with a participant “retaliating” by electing to reject an unfair financial offer, despite the fact that this choice was “costly” as both the participant and the offerer would not receive any money. Furthermore, they described that acute lowering of central serotonin levels with dietary tryptophan depletion led participants to reject moderately unfair offers more frequently, and take longer to accept the fairest offers. Activity of the dorsal striatum increased during rejections to a degree commensurate with the increased frequency of rejecting offers [61]. These findings of an interaction between serotonergic modulation and dorsal striatal activity in punitive, albeit costly, responses to unfair social exchanges is consistent with a finding from a recent study of ours, in which we observed that lower striatal serotonin transporter levels were related to greater state-levels of aggression in personality disordered patients [64].
Neurochemistry and Pharmacology: Serotonin
Initial studies of serotonin and aggression
One of the best studied areas of the neurobiology of aggression is the role of the serotonergic system. The first evidence for a role for serotonin (5-HT) in aggression came from animal models, in which isolation-induced aggression was shown to be reduced by numerous 5-HTergic antagonists and by pharmacological 5-HT depletion [65]. Subsequent evidence from human studies revealed a negative relationship between cerebrospinal fluid (CSF) 5-hydroxyindoleacetic acid (5-HIAA), the major metabolite of 5-HT catabolism, and aggression in patients with personality disorders [66,67]. The inverse correlation between 5-HIAA and trait aggression was interpreted as a hypo-serotonergic mechanism of impulsive aggression.
Further early findings demonstrated altered platelet 5-HT parameters, such as decreased 5-HT uptake in aggressive compared to non-aggressive participants [68] and decreased platelet imipramine binding (a measure of 5-HT transporter binding) in aggressive children with conduct disorder [69]. Attenuated hormonal responses to 5-HTergic pharmacological challenges also contributed to the initial foundation of the 5-HT hypothesis of aggression [70–72]. Finally, the selective serotonin reuptake inhibitor (SSRI) fluoxetine was shown to have an anti-aggressive therapeutic effect in personality disordered patients that was independent of antidepressant or anxiolytic effect [73,74].
Studies of 5-HT CSF metabolites, platelet measures, hormonal responses to pharmacological challenge, and the anti-aggressive effect of fluoxetine have been repeated and re-examined. There has been significant variability with respect to CSF 5-HIAA levels. While the classic finding consists of an inverse relationship [66,67,75], at least one study described a positive correlation [76], whereas another revealed no covariance, despite a reduced fenfluramine-induced prolactin response (and index of presynaptic 5-HT) in the same cohort [77]. While platelet 5-HT concentration [78] and 5-HT2A receptor levels [79] have also been shown to be related to aggression, reduced platelet 5-HTT binding site number in aggressive patients appears to be the most consistent platelet finding [80]. It is worth noting, however, that at least two earlier studies found a positive relationship between 5-HTT platelet binding sites and aggression [81,82]; however, these studies were not done in as well-defined an aggressive population, whereas those more consistently demonstrating reductions in platelet 5-HTT have been performed in impulsive aggressive personality disordered patients either with or without a formal diagnosis of IED.
Reduced fenfluramine-induced increases in plasma prolactin also appears to be a highly reliable finding in aggression [70–72,77,83]. Change in serum prolactin after fenfluramine challenge is negatively related to trait aggression, and is lower in personality disordered patients with the more recent, integrated research criteria for IED (IED-IR); this effect is abrogated when covarying for differences in trait aggression. Moreover, prolactin level changes were not related to impulsivity; a diagnosis of BPD or ASPD; nor mood, anxiety, or alcohol/substance abuse disorders [26]. Therefore, these findings provided support for a specific involvement of 5-HT in aggression.
While these findings discussed above established the importance of the 5-HT system in aggression, further studies have been essential in more directly demonstrating the ability of the 5-HT system to modulate aggressive behavior, and address questions related to molecular genetics and pharmacology, development, and neuroanatomy. In the following sections, we will discuss the literature related to these issues.
5-HT challenges in humans: laboratory aggression, and functional neuroimaging
One of the primary methods for investigating the role of the 5-HT system in aggression is by the use of peripherally administered agents that acutely attenuate or enhance central 5-HTergic function. One of the most common and well-described interventions is dietary depletion of tryptophan, the amino acid precursor of 5-HT biosynthesis, which has been validated as a means to rapidly lower central 5-HT [84–86].
Early studies demonstrated that tryptophan depletion increased aggressive responding in nonclinical male participants in laboratory measures of aggression, which is consistent with the low-presynaptic 5-HT hypothesis [87]. However, it has become increasingly apparent that the relationship between 5-HT and aggression is complex, as a variety of factors appear to moderate how challenges to the 5-HT system affect aggressive responding.
Using a laboratory aggression paradigm, Bjork et al. [88] found that tryptophan depletion and tryptophan loading (which enhances central 5-HT availability) increased and decreased the frequency of aggressive responses, respectively, in those with high trait aggression. In those with low trait aggression, on the other hand, the effect was reversed, with decreases and increases in aggressive responding occurring under tryptophan-depleted and -loaded conditions, respectively [88]. Similarly, Kramer et al. [89] described an attenuation of aggressive responding with tryptophan depletion in a laboratory aggression paradigm, but only in those with low trait aggression, with no effect on those with high trait-aggression levels. In studies of adults and adolescents with ADHD, tryptophan depletion increased aggressive responding in a laboratory paradigm, but only in those with low trait impulsivity [90–92].
In addition to depletion/loading of dietary tryptophan, studies have also employed acute administration of SSRIs as a means to temporarily heighten synaptic 5-HT. Two studies have demonstrated that the delivery of severe, retaliatory, (laboratory-based) aggressive responses (which occurs only in those with either high levels of primary psychopathy [93] or significant histories of aggression [94], under levels of high-provocation) were reduced, acutely, after a single dose of an SSRI.
A handful of studies have begun to examine the neurobiological mechanisms involved in 5-HTergic modulation of impulse control and emotion processing relevant to aggression. Right orbitofrontal activity during inhibition of a motor response (i.e., the no-go condition of a go/no-go task) was reduced after tryptophan depletion [95]. In a laboratory aggression paradigm, tryptophan depletion was associated with reduced insula activity during selection of severity of aggressive response [89]. Acute challenges to the serotonergic system have also been shown to influence the processing of emotional faces [96] and associated neural activity. Tryptophan depletion modulated the functional connectivity that occurs between the amygdala and prefrontal regions specifically while processing angry faces, as opposed to neutral or sad faces [59].
Last, Grady et al. [97] compared the effects of attenuated and enhanced central 5-HT availability (with tryptophan depletion and acute citalopram administration, respectively) on the processing of neutral, angry, and fearful faces. First, they found two separate networks –one that was selective for fearful faces relative to angry and neutral expressions, and the other that distinguished between fearful and neutral faces, but with responses to angry faces that were intermediate to those of neutral and fearful ones. In both networks, tryptophan depletion specifically enhanced responses to angry faces, such that they were more like the responses to fear than neutrality. Citalopram treatment, on the other hand, led to neural network responses that were equivalent across the three emotions, yet by differing mechanisms. In the first network, increased 5-HT with citalopram decreased responses to fear equal to that of responses to neutrality. In the second network, on the other hand, citalopram increased responses to both neutral and angry faces to be similar to that of fear.
In summary, studies of acute manipulations of 5-HT availability in humans have provided strong support for a modulatory role of aggression by the 5-HTergic system. The mechanism by which 5-HT influences aggression is likely through neural networks involving the amygdala, ventro-medial prefrontal regions, and the striatum. These findings also make clear that the relationship between 5-HT and aggression is not a simple, linear function, and trait dimensions (namely aggression, impulsivity, psychopathy) and the quality of aggression (normal, pathological, reactive vs. proactive) are important moderating variables. The neural basis of the complex relationship between 5-HT and aggression likely owes to parallel neural circuits that are modulated by 5-HT, yet are differentially involved in the various facets of appraisal and response to social threat-stimuli.
Molecular biology of the 5-HT system in aggression: genetic, pharmacological, and neuroimaging studies
A wide variety of studies, methodologically speaking, have identified various molecular components of the 5-HT system involved in aggression, which can be broadly categorized as being involved in either 5-HT metabolism/turnover or 5-HT receptor signaling. Much of this work has been performed in animal genetic and pharmacological studies; however, there are also data from human genetic studies and neurochemical imaging.
5-HT synthesis and turnover: tryptophan hydroxylase-2
Tryptophan hydroxylase-2 (tph2) was recently found to be the brain-specific, rate-limiting enzyme mediating 5-HT synthesis [98]. The mouse tph2 single nucleotide polymorphism (SNP) C1437G has been associated with differential rates of 5-HT synthesis, and was used as a model to examine aggressive behavior, as well as depression and anxiety. Mice homozygous for the high-activity tph2 allele (1437C) were shown to exhibit greater levels of aggression compared to mice homozygous for the low-activity allele (1437G) [99]. Similarly, in a study in which highly aggressive mice were bred with a less aggressive laboratory breed, midbrain tph2 mRNA was shown to be positively correlated with the extent of severe aggression in certain resultant strains [100]. While these two former studies indicate that greater tph2 activity results in higher levels of aggression, mice with both tph2 copies deleted or “knocked out” (tph2 − / − ) were found to be more aggressive than tph2( − / + ) and tph2 ( + / + ) mice [101]. Therefore, again, we see that the contribution of the 5-HT system to aggression is complex: “low” tph2 activity and “no” tph2 activity both affect aggression, albeit, in a contrasting manner. This is likely due to differential developmental pathways that occur in the absence of functional protein, and the development of qualitatively different forms of aggression.
To our knowledge, the human equivalent of a null tph2 mutation has not been identified. However, “risk” tph2 polymorphisms have been described in humans and nonhuman primates. Homozygosity for a functional tph2 SNP (associated with altered morning, basal cortisol levels) in rhesus monkeys was associated with attenuated aggressive behavior; however, this effect depended on a gene x environment interaction, as the anti-aggressive effect of this allele occurred only in those animals maternally reared, as opposed to being raised by peers [102]. Polymorphisms of tph2 have also been linked to anger-related personality traits [103,104]; Cluster B and C personality disorders; and the personality traits harm-avoidance [105] and reward dependence [106]. Our group recently found that a risk tph2 haplotype occurred with greater frequency in patients with BPD compared to healthy controls, and BPD patients with the risk haplotype exhibited greater levels of aggression, as well as affective lability and suicidal/parasuicidal symptoms [107].
Polymorphisms of tph2 have also been related to structural and functional neuroanatomical differences related to aggression. Differential bilateral, dorsal amygdala reactivity to processing of angry/fearful faces (a putative biomarker of aggression) was shown to be related to tph2 polymorphism genotype [108]. In vivo 5-HT synthesis capacity, specifically, in the medial OFC – a brain region that plays a key role in aggression – has been shown to be related to tph2 polymorphisms in healthy, human control participants [109]. Moreover, carriers of a risk tph2 allele had larger medial OFC volumes [100], as well as reduced amygdala and hippocampal volumes [102].
5-HT synthesis and turnover: 5-HT transporter
The 5-HT transporter (5-HTT) is distributed along the axons and synaptic terminals of 5-HTergic neurons and plays an essential role in the clearance of extracellular 5-HT. There is a considerable mouse and human genetics literature on the role of the 5-HTT in aggression.
Attenuated aggression, as well as increased anxiety and stress-responses, were observed in mice with both 5-HTT genes knocked out (KO) [110,111]. Importantly, there appear to be interactions between genotype and the context of aggression. Homozygous 5-HTT KO mice were less aggressive than wild-type and heterozygous KO mice, specifically when in the opponent’s environment as opposed to either a neutral or home setting. Heterozygous 5-HTT KO mice, on the other hand, were more aggressive than wild-type mice in their home environments, but no different than wild-type mice in a neutral setting or that of the opponent. Aggression was not affected by venue (home, neutral, or opponent’s) in the wild-type. Last, no differences in aggression were found among the three genotypes in a neutral environment [110].
Mouse studies of genetically deleted 5-HTT have been complemented by those employing pharmacological antagonism of the 5-HTT (e.g., with fluoxetine treatment), which has allowed for the isolation of specific developmental periods. Interestingly, aggression in mature mice is reduced when mice are treated with fluoxetine during either an early postnatal period or a peri-adolescent one; increases in anxiety in the mature mouse, however, occur with fluoxetine treatment only during the early postnatal period, but not peri-adolescence [112]. Therefore, the developmental roles of 5-HTT in aggression and anxiety are not necessarily fully interdependent, particularly during the critical peri-adolescent period. Surprisingly, it was demonstrated that male offspring of mice treated with fluoxetine while pregnant (i.e., in utero 5-HTT inhibition) exhibited increased aggression and reduced anxiety [113], which, remarkably, is the opposite effect of postnatal fluoxetine treatment. Therefore, in addition to critical, early postnatal, and peri-adolescent developmental periods, in which 5-HTT antagonism lessens aggression in the adult, a separate, prenatal period may exist during which 5-HTT antagonism leads to increased aggression in the mature mouse.
Human genetic studies have also examined the role of the 5-HTT in aggression. The most common 5-HTT gene variant consists of the “short” and “long” forms of the 5-HTT promoter region (conventionally referred to as the 5-HTT-linked polymorphic region or 5-HTTLPR), which are associated with relatively decreased and increased expression, respectively [114]. Although at least one study, of adult males with intellectual disabilities, demonstrated an association between the long 5-HTTLPR allele and greater levels of aggression [115], the short allele has more consistently been shown to confer risk for aggression and violence in a range of populations [116–118]. Furthermore, it appears that the contribution of the short 5-HTTLPR allele to increased aggression is moderated by environmental factors, such as acute stress [119], recent, chronic stress [120], and childhood adversity [121].
In addition to the 5-HTTLPR, another risk polymorphism consists of a variable number of tandem repeats (VNTR) within intron 2 of the 5-HTT gene (STin2). The two major variants of STin2 consist of a 10- and 12-repeat, with the latter being the risk allele. Two studies have demonstrated that the contribution of risk by the 5-HTT gene for disinhibited/impulsive behavior [122] and aggression [123] was most discernible when both the 5-HTTLPR and STin2 polymorphisms were taken into account (namely, at least one short 5-HTTLPR allele and two STin2 12-repeat alleles).
In addition to assessing genotype, studies have begun to characterize the epigenetic status of the 5-HTT gene. Generally speaking, such studies have demonstrated a relationship between differential 5-HTT gene methylation and 5-HTT mRNA levels [124], vulnerability to PTSD [125], differences in depressive symptoms between monozygotic twins [126], hippocampal gray matter volume [127], and amygdala threat-related activity [128]. At least one study has demonstrated greater 5-HTT gene methylation in adults with a history of childhood aggression compared to adults without such a history; furthermore, in vivo brain 5-HT synthesis in the OFC was negatively correlated with 5-HTT gene methylation [129].
Neurochemical imaging studies, i.e., those employing PET or SPECT, have also been performed with radioligands with high affinity for 5-HTT. An early PET study of ours revealed lower 5-HTT binding in the left ACC in impulsive-aggressive personality disordered patients [130], which suggests attenuated presynaptic 5-HT in frontolimbic regions and, therefore, is consistent with the hyposerotonergic model of impulsive aggression. In a more recent study, employing a more specific radioligand and larger cohorts of personality disordered IED patients and healthy controls we did not observe the predicted inverse relationship between ACC 5-HTT availability and trait aggression until we statistically adjusted for trait callousness (a measure of the interpersonal/affective component of psychopathy), which itself was positively correlated with ACC 5-HTT availability [64].
5-HT synthesis and turnover: MAOA
Monoamine oxidase A (MAOA) is a catabolic enzyme present in the outer mitochondrial membrane of synaptic terminals of monoaminergic projection neurons; its major neurochemical substrates are 5-HT, NE, and to a lesser extent DA [131]. MAOA was one of the first genes identified as playing a role in the heritability of impulsive aggression, as a rare missense mutation was identified in a Dutch kindred with a significant history of impulsive aggression [132]. A more common human polymorphism of MAOA, consisting of a VNTR in the promoter region, has also been well characterized [133]. Carriers of the low-expressing MAOA-VNTR allele (3 repeats) exhibit greater aggression compared to those with the high-expressing allele (3.5 and 4 repeats) [134,135], and the low-expressing allele occurs more commonly in violent compared to nonviolent incarcerated males [136]. Moreover, numerous studies have demonstrated a gene x environment (GxE) effect, such that greater early-life stress increases the risk for impulsive aggression in males with the low-expressing MAOA-VNTR allele (note that this effect is most robust in men, as MAOA is an X-linked gene) [137]. This GxE effect has also been demonstrated in nonhuman primates [138,139]. Finally, using PET imaging with the MAOA radioligand [11C] clorgyline, trait aggression in a healthy, nonclinical male population was negatively associated with brain MAOA availability in cortical and subcortical regions; furthermore, this effect was independent of MAOA-VNTR genotype [140].
Mouse genetic and pharmacological studies have helped to clarify the pathogenesis of MAOA-associated aggression. Increased aggression has been observed in both MAOA knockout mice [141] and mice with a naturally occurring mutation [142] that very closely mimics the rare missense mutation of the Dutch kindred (resulting, effectively, in a naturally occurring knockout). Early studies demonstrated that brain 5-HT levels, compared to NE and DA, are preferentially increased in the early postnatal phase of mice with MAOA gene deletions, thus suggesting that the increased aggression associated with loss or attenuation of MAOA activity was 5-HT-dependent [141]. However, while inhibition of 5-HT synthesis in the early postnatal period of MAOA deficient mice has been shown to abrogate certain neuroanatomical and behavioral changes [143], the development of heightened aggression, nevertheless, is unchanged [144]. Furthermore, inhibition of the dopamine transporter, but not the 5-HTT or the norepinephrine transporter, in developing mice leads to an aggressive phenotype comparable to that of MAOA deficient mice [112]. Therefore, a question for future studies will be to clarify the role of excessive 5-HT vs. DA in the pathogenesis of MAOA-dependent aggression.
Receptor subtypes: 5-HT1A
The 5-HT1A receptor plays a clear role in mood and anxiety disorders [145], and there is a growing literature examining its role in aggression, as well as the closely related construct of impulsivity. The 5-HT1A receptor is expressed on two neuronal populations: (1) as an autoreceptor, in a somato-dendritic distribution, on raphe serotonergic neurons; and (2) postsynaptically, on non-serotonergic hippocampal, septal, and cortical neurons. In both populations, the 5-HT1A receptor promotes membrane hyperpolarization, and therefore, mediates an inhibitory action on target cells [146].
In various rodent models, pharmacological treatment with 5-HT1A agonists attenuates aggressive behaviors [147,148]; there is evidence for the importance of actions at both raphe autoreceptors and frontal postsynaptic receptors [149–152]. There is differential 5-HT1A receptor expression and 5-HT1A-dependent functional responses in rodent models of aggression [153–156]. In 5-HT1A receptor knockout mice, there is little to no evidence of altered aggression, despite a clear effect on measures related to mood and anxiety conditions. On the other hand, however, mice overexpressing the 5-HT1A receptor (specifically in raphe, 5-HTergic neurons) exhibited heightened aggression [157]. Importantly, this effect was dependent on overexpression of the 5-HT1A receptor during adulthood rather than developmentally.
In humans, agents with significant 5-HT1A receptor activity, e.g., buspirone, have not played a significant role in the pharmacotherapy of aggression and violence. Nevertheless, a consistent finding has been an inverse relationship between 5-HT1A receptor-dependent increases in serum cortisol and trait aggression in personality disordered patients [70,158,159]. In healthy participants, however, 5-HT1A receptor-dependent increases in serum cortisol were positively correlated with trait aggression, suggesting differential involvement of 5-HT1A receptor function in pathologic and “normal” forms of aggression. Polymorphisms of the 5-HT1A receptor gene have not been shown to be involved in impulsive aggression; however, they have been implicated in trait impulsivity [160] and BPD [161]. Curiously, 2 PET studies, both using the same 5-HT1A receptor radioligand ([11C]WAY100635) in healthy participants, demonstrated the opposite relation between frontal 5-HT1A receptor availability and trait aggression. One showed a positive [162] and the other an inverse correlation [163]. We speculate that the role of the 5-HT1A receptor in aggression may be due to pathophysiologic heterogeneity.
Receptor subtypes: 5-HT1B
The 5-HT1B receptor is expressed in raphe 5-HTergic neurons, as well as non-5-HTergic (namely GABAergic and glutamatergic) projection neurons originating from the striatum and hippocampus (namely, CA1 pyramidal cells). The receptors themselves are localized primarily at axonal terminals, and are believed to regulate neurotransmitter release [164]. Multiple rodent studies have demonstrated the ability of 5-HT1B agonists to mediate an anti-aggressive effect; however, which neuronal populations are responsible for this effect remains a matter of debate [150,165–172]. Mice with both copies of the 5-HT1B receptor gene knocked out have been shown to exhibit enhanced aggression [173] as well as greater impulsivity [174]. In humans, evidence for a role of the 5-HT1B receptor in aggression has been limited to genetic polymorphisms [175–178]. No PET imaging studies have been performed examining 5-HT1B receptor availability in aggression; however, such studies have been performed in externalizing or impulse control disorders, such as pathological gambling and alcohol dependence. In pathological gambling, 5-HT1B availability in the striatum and anterior cingulate was positively correlated with severity of gambling [179], and 5-HT1B availability was greater in the ventral striatum in patients with alcohol dependence compared to controls [180]. In major depression, on the other hand, 5-HT1B binding is decreased in the ventral striatum compared to controls [181]. Thus, there appear to be contrasting differences in 5-HT1B availability in impulse control disorders (increased 5-HT1B) compared to an “internalizing” condition (decreased 5-HT1B) such as major depression.
Receptor subtypes: 5-HT2A
Consistent with the important anti-aggressive and mood stabilizing effects of agents with 5-HT2A receptor antagonist activity, the 5-HT2A receptor has also been the focus of studies examining the etiology, pathogenesis, and pathophysiology of impulsive aggression. In animal pharmacological studies, the 5-HT2A receptor has been shown to promote impulsive and aggressive behavior [182–184]. A number of human genetic studies have revealed an association between 5-HT2A receptor polymorphisms and aggression [185] and impulse control disorders [186–191]. In a postmortem study of suicide victims, prefrontal 5-HT2A receptor binding was positively correlated with lifetime history of aggression [192].
A number of PET imaging studies have revealed an association between the 5-HT2A receptor and aggression and impulsivity; however, the results have been conflicting. We recently found that 5-HT2A receptor availability in the OFC was positively associated with state levels of aggression in a physically aggressive personality disordered (predominantly BPD) population meeting criteria for IED [58]. This finding was consistent with our view that OFC 5-HT2A receptor activity promotes aggression, in a dynamic, state-dependent manner, in those with IED. We suspect that increased OFC 5-HT2A receptor availability may be due to psychosocial stress [193], and that greater 5-HT2A receptor function in the OFC may promote aggression by decreasing amygdala–OFC coupling that occurs during threat processing [57]. At least two other studies found, however, that frontal 5-HT2A receptor availability was negatively correlated with trait aggression [194] in patients with BPD, and impulsivity [195] in a highly assaultive population with ASPD. Yet another study found no differences in cortical 5-HT2A receptor levels between high- and low-aggressive participants, as well as no relation to trait aggression or impulsivity [196].
These studies did not use a measure of state aggression and did not assess for whether participants met criteria for IED; therefore, it is difficult to compare these findings with our study. However, we suspect that the negative correlation between trait aggression and impulsivity and cortical 5-HT2A receptor availability may reflect a compensatory, albeit incomplete, downregulation of the 5-HT2A receptor in aggressive/impulsive individuals.
Receptor subtypes: 5-HT3
The 5-HT3 receptor is the only 5-HT receptor that functions as a ligand-gated ion channel, whereas all other known 5-HT receptors are G-protein coupled. The 5-HT3 receptor has both a pre- and postsynaptic distribution, and in the forebrain is expressed at least in part by a subpopulation of interneurons [197]. One 5-HT3-dependent mechanism of particular relevance to impulsivity and aggression is the regulation of mesolimbic dopamine release [198], which plays a key role in motivation and reward. The possibility that the 5-HT3 receptor is involved in impulsive aggression is limited to animal pharmacological studies, in which 5-HT3 receptor antagonists and agonists led to attenuated and increased aggressive behavior, respectively [199–203]. To our knowledge, no clinical studies have examined 5-HT3 receptor antagonists in aggression. In alcohol use disorders, however, 5-HT3 antagonism has been shown to lessen alcohol consumption [204], lessen subsyndromal mood symptoms [205], and attenuate alcohol cue-induced dopamine release in the ventral striatum [206]. Genetic polymorphisms of the 5-HT3 receptor have been associated with impulse control disorders, such as comorbid alcoholism and ASPD [207], and the pathological personality traits of harm avoidance and nonconformity [208]. Furthermore, 5-HT3 polymorphisms are associated with differential structure and function of limbic and prefrontal regions [209,210]. Future studies are needed to examine 5-HT3 antagonists clinically in aggressive populations, however.
Neurochemistry and Pharmacology: Dopamine
In contrast to the serotonergic system, much less has been described regarding the role of the dopaminergic (DAergic) system in aggression. Nevertheless, the DAergic system is poised to play an important role in aggression, given its involvement in decision making, reward, motivation, and higher-order cognitive processes. Additionally, the DAergic system is involved in the pathophysiology and psychopharmacology of conditions such as ADHD and schizophrenia, which commonly involve aggressive behavior.
A recent study by Schlüter et al. [211] is one of the first to use neurochemical imaging to specifically examine the role of the DAergic system in aggression in humans. Dopamine synthesis and storage capacity were assessed with PET imaging using [(18)F]DOPA in a cohort of healthy, nonclinical men who engaged in a computer-based laboratory task designed to assess aggressive reactions in response to provocation by an unseen and fictitious counterpart.
It was found that the frequency of aggressive responses by participants was inversely correlated with DA synthesis capacity, most notably in the midbrain, but also in the striatum. Additionally, DA storage capacity in the midbrain was negatively correlated with aggressive responses. These findings suggest that greater availability of dopamine may protect against non-advantageous, aggressive responses to provocation. Important questions that remain are (1) how do these findings relate to pathological forms of aggression occurring in a “real world” or non-laboratory setting, and (2) which “down-stream,” DA-modulated neuroanatomical regions are being affected and leading to differential aggressive responses?
As studies of dopaminergic neurochemistry and aggression are currently limited, comparison with findings of related constructs, such as psychopathy, may also be informative. Buckholtz et al. [212] assessed presynaptic DA release in a community sample of adults without a history of substance abuse, who were characterized with multiple self-report measures of various personality dimensions, including the Psychopathic Personality Inventory (PPI). Presynaptic DA release in the nucleus accumbens, a striatal region that plays a critical role in reward, was found to be positively correlated with the “impulsive-antisocial” component of the PPI; impulsive antisociality is related to, but is somewhat broader than, impulsive aggression. No relation between accumbal presynaptic dopamine was found with the “fearless dominance” component of the PPI, which consists of fearlessness, social influence, and stress immunity. Moreover, the relation between presynaptic DA release and impulsive antisociality remained significant even after adjusting for measures of impulsivity, extraversion, and novelty seeking.
Thus, there is an apparent conflict between the studies by Schlüter et al. [211] and Buckholtz et al. [212], with the former indicating a negative relationship between presynaptic DA and aggression and the latter suggesting the opposite. We suspect that the findings of the Schlüter et al. study may reflect the cognitive enhancing effects of adequate DAergic availability on fronto-cortical systems, which may mitigate maladaptive, aggressive responses to frustration. The increased presynaptic DA release in the ventral striatum observed in the Buckholtz et al. study, however, may reflect aberrant regulation at synaptic DA terminals in the ventral striatum, and therefore impairment of reward-related processes.
A somewhat broader literature exists regarding the molecular genetic basis of the role of the dopaminergic system in aggression. A number of studies have examined the role of catechol-O-methyltransferase (COMT), which is involved in the catabolism of DA as well as other catecholamines such as norepinephrine. The Val158Met polymorphism is a common and well-studied variant of COMT: the Met allele is the lower activity variant, resulting in higher basal levels of synaptic dopamine. Interestingly, in patients with BPD, a history of childhood sexual abuse was associated with lower levels of trait aggression, but only in those homozygous for the higher activity, Val158 COMT allele [213]. This suggests that early psychosocial adversity in those with low basal DA availability may attenuate trait aggression, at least in those predisposed to develop BPD. In patients with schizophrenia, the low activity, Met158 COMT allele has been shown to increase the risk of aggression and violence [214]. While not all studies investigating the Val158Met COMT polymorphism have demonstrated its involvement in aggression [215–217], less well-described COMT variants have been implicated in violent offenders with antisocial personality disorder [217], aggressive personality disordered patients [215], and highly aggressive children [218]. Questions for future studies are (a) to what extent is differential COMT activity involved in aggression during development as opposed to adult brain function, and (b) which catecholaminergic substrate of COMT is predominantly involved in determining aggression – DA, or others, such as norepinephrine?
At least two studies have demonstrated a role for the 48 bp repeat exon III polymorphism of the dopamine D4 receptor in aggression. The 7-repeat (7R) D4 polymorphism was found to be significantly more common in aggressive patients with schizophrenia, compared to non-aggressive schizophrenia patients and healthy controls [219]. Moreover, D4 7R allele carriers exhibited significantly greater “physical aggression against others” compared to the non-7R carriers; however, no differences in 7R allele frequency were observed with respect to other forms of aggression, namely “verbal aggression,” “self-directed aggression,” and “physical aggression against objects.” Such a finding suggests the possibility, for example, that the D4 receptor could be related to proactive aggression, which consists of greater physical assaultiveness. In a separate study, a significant gene x environment interaction was found with the D4 receptor, such that increasing levels of prenatal stress were associated with higher levels of trait aggression manifested during young adulthood only in those with the 7R D4 allele [220]. Neither prenatal stress nor the 7R D4 allele alone was related to trait aggression during young adulthood. Moreover, this effect was specific for prenatal stress, as maternal stress up until the age of 15 of participants was not related to aggression, alone or as a function of D4 allele frequency.
These findings indicate that the DAergic system, in terms of gene x environment interactions, may be involved during development in determining an individual’s propensity for aggression. Issues to be addressed in future studies include (a) whether DAergic agents, such as those targeting the D4 receptor, might have anti-aggressive therapeutic potential in the adult, and (b) whether aggression mediated by the DAergic system differs qualitatively from aggression related to 5-HTergic abnormalities.
Neurochemistry and Pharmacology: Vasopressin
While the arginine vasopressin (AVP) system has been well characterized in terms of its neuroendocrine role in the cardiovascular and renal systems, a significant number of animal studies have revealed its role as a direct neuromodulator in the CNS that regulates various social behaviors, such as aggression. Early studies in the hamster demonstrated that direct, hypothalamic administration of AVP enhanced aggressive responses [221,222], whereas AVP antagonists attenuated them [223]. More recent studies in a rat model of maternal aggression revealed that AVP release in the central nucleus of the amygdala was increased during maternal aggression, and the degree of AVP release was positively correlated with the degree of aggressive behavior. Furthermore, bilateral central amygdala administration of a vasopressin 1a receptor antagonist attenuated maternal aggression, and in a related rat strain with low levels of maternal aggression, AVP administration into the central amygdala nucleus increased aggression [224].
In mouse genetic models, knockout of the AVP 1b receptor reduced aggression [225,226], whereas deletion of the 1a receptor did not [227], despite the fact that pharmacological manipulations at the 1a receptor significantly influenced aggression and other social behaviors in animal models. A rat line with a naturally occurring deletion of the vasopressin gene also demonstrated decreased aggression and impulsivity [228].
Human studies have also lent support to the role of the AVP system in impulsive aggression. In a study of impulsive aggressive personality disordered patients, AVP obtained from the CSF was positively correlated with trait aggression, and negatively correlated with a measure of central 5-HTergic activity (i.e., fenfluramine-induced serum prolactin levels) [77]. The positive relation between CSF AVP and trait aggression persisted after covarying for fenfluramine-induced serum prolactin (an index of 5-HT activity), indicating that the relationship between AVP and aggression cannot simply be accounted for by the relationship between AVP and 5-HT. Intranasal administration of AVP has been shown to affect emotion processing in humans [229,230], and the orally available AVP V1a receptor antagonist, SRX246, was shown to block the effect of intranasal AVP on the neural responses to angry faces in the right amygdala and various other cortical regions associated with social information processing [231]. Last, a handful of human genetic studies have suggested that AVP receptor polymorphisms are associated with human aggression, particularly, the 1b receptor gene and childhood aggression [232–234].
Therefore, both genetic and pharmacological evidence in animal models and humans suggests that AVP may promote aggression in regions such as the central amygdala, and this effect may be mediated by the V1a and/or V1b receptors. Furthermore, pharmacological treatments that target the AVP system may be a novel therapeutic modality for aggressive behaviors.
Steroid Hormones: Cortisol and Testosterone
We will examine the steroid hormones cortisol and testosterone together, as there is growing evidence that these two steroid hormones may contribute to aggression and violence in an interdependent manner. Initial studies, of male adolescents with disruptive/delinquent behaviors, revealed a relationship between low baseline cortisol and heightened trait aggression [235]. Subsequently, it was found that a positive correlation between serum testosterone levels and aggression depended on the presence of low serum cortisol [236]. Thus, the effect of testosterone on aggression appeared to be moderated by serum cortisol levels.
More recent studies have revealed that the interaction between cortisol and testosterone may be more complex than a “high testosterone/low cortisol” model, and we suspect this may be related to gender and whether participants are either healthy/nonclinical, or are highly aggressive and/or exhibit psychopathic traits. For example, in nonclinical participants, trait aggression was positively associated, only in men, in those with either (a) high levels of testosterone in conjunction with high SSRI-induced cortisol, or (b) low testosterone and low SSRI-induced cortisol [237]. In a separate study, also in nonclinical participants, subclinical psychopathic traits was positively related to testosterone levels in those with high cortisol, and conversely, in those with low cortisol, subclinical psychopathy was negatively related to testosterone levels; both of these effects were only observed in the male participants [238]. In a study of a nonclinical group of women, aggressive responses after provocation in a laboratory setting were greater either in those with high testosterone/high cortisol or low testosterone/low cortisol [239]. Last, within a criminal population, morning cortisol levels were negatively correlated with psychopathy scores in psychopathic criminals, but not nonpsychopathic ones [240]. Therefore, while testosterone and cortisol appear to be inter-related with respect to aggression, the nature of this relationship likely differs as a function of variables such as gender, psychopathy, and other pathological personality dimensions.
Studies have begun to reveal potential neurobiological mechanisms that underlie the effect of cortisol and testosterone on aggression. Derntl et al. [241] demonstrated that reaction times to recognizing fearful male faces, specifically, was negatively related to morning testosterone levels in a nonclinical male population. In other words, greater testosterone levels were associated with recognizing fearful male faces more quickly. Notably, no relationship between testosterone and reaction times to recognizing other emotional facial expressions was observed [241], indicating that this effect was specific to the fear-processing circuitry associated with aggression, rather than a more global effect on facial affect processing. Amygdala responses to angry and fearful facial expressions, specifically, were directly correlated with testosterone levels [241], thus implicating the amygdala fear-processing circuitry as a potential substrate of the aggression-promoting effects of testosterone.
Hermans et al. [242] found that in a group of nonclinical, female participants, the degree of activation of the amygdala, hypothalamus, and brainstem while viewing angry faces was directly related to the baseline testosterone-to-cortisol (T/C) ratio. Interestingly, while the lateral OFC was also activated in response to angry faces in this study, its activity was not related to the T/C ratio, indicating that contribution of these steroid hormones may act on specific anger-associated brain circuits. In a nonclinical group of women, van Wingen et al. [243] found that intranasal testosterone administration reduced functional coupling of the left amygdala with the left OFC and with the right amygdala while performing a facial emotional processing task, indicating that testosterone may modulate amygdala–PFC circuitry involved in social threat processing. Finally, exogenous testosterone administration, after first normalizing endogenous testosterone with treatment with a gonadotropin releasing hormone antagonist in a group of nonclinical males, was associated with increased amygdala, hypothalamus, and periaqueductal gray activity, compared to placebo, while viewing angry facial expressions [244].
Collectively, these findings suggest that cortisol and testosterone influence aggression and related factors such as psychopathy in an interdependent manner; however, this relationship is complex, and likely depends on factors such as age, gender, and degree of trait aggression and psychopathy. Cortisol and testosterone likely influence aggression and psychopathy through the modulation of amygdala–PFC fear or threat circuitry. Areas of focus for future studies consist of identifying the precise loci of action of these steroid hormones, as well as the underlying cellular, molecular, and physiologic effects.
Conclusion
The studies we examined in this review have enhanced our understanding of the neurobiology of aggression and violence, but have also revealed areas of inconsistency that require clarification, as well as clues for avenues of future inquiries.
A relatively consistent and specific finding has been an association between reduced amygdala volumes and greater levels of trait aggression. Optimal resolution of this relationship appears to require analysis of a number of additional variables, such as amygdala subregion and hemisphere, aggression subtype (reactive vs. proactive), and covariant dimensions (namely impulsivity, emotional reactivity, and psychopathy). Inconsistencies between studies – such as some demonstrating reduced whole amygdala volumes, bilaterally, and others indicating a specific involvement of the left dorsal amygdala – likely are resolvable by accounting for these anatomical and phenomenological factors. Therefore, it is likely that different processes (namely perceptual vs. motor; effortful vs. habitual) of different aggression subtypes are a function of amygdala subregion and hemisphere.
In terms of functional abnormalities, pathological aggression is associated with a more labile range of amygdala activity, as well as differential amygdala responsivity to socially threatening stimuli. Commonly, aggression is associated with heightened amygdala reactivity to socially threatening stimuli (e.g., fearful or angry faces), and, remarkably, responses to neutral interpersonal stimuli (e.g., neutral facial expression) are also heightened. Amygdala hyporesponsiveness to threat, however, has also been observed, and this may be related to elements of aggression that are driven by the interpersonal/affective dimensions of psychopathy. Similar to the inconsistencies of amygdala volumetric changes, disentangling the role of amygdala hyper- vs. hyporesponsiveness to interpersonally salient cues will also likely require resolving the amygdala into its component functional subregions and characterizing aggression subtypes and associated dimensions. Furthermore, the 5-HTergic system also appears to be involved in determining amygdala responses to neutral and aversive interpersonal stimuli, and this effect may also be subregion- and circuit-dependent.
An important issue that remains unexamined relates to the cellular and ultrastructural basis of amygdala volumetric alterations, as well as the mechanism by which such changes yield the above-described functional differences. Animal models suggest that morphological alterations in the amygdala may reflect decreased dendritic arborization of excitatory [245–248] and local inhibitory neurons [249], which could therefore disrupt both “top-down” cortical inhibition as well as local inhibitory processes. As stress hormones or glucocorticoids are involved in dendritic remodeling in limbic regions in animal models, a potential avenue for future studies may be determining whether the cortisol abnormalities observed in aggressive individuals are related to amygdala structural changes. Such a line of inquiry would also have the potential for developing therapeutic strategies, such as those that involve pharmacological manipulation of the hypothalamic–pituitary–adrenal axis.
The OFC and ACC are limbic prefrontal regions that also play a key role in the neurobiology of aggression, particularly in terms of their interconnectivity with the amygdala. Involvement of the OFC and its coupling with the amygdala likely impairs (a) the ability to ascribe affective and motivational significance to stimuli in a manner that is integrated, moderated, and flexible; and (b) “top-down” modulation of central nucleus output, leading to an increased likelihood of a “visceral/ sympathetic” component to a stimulus representation. Altered coupling between the ACC and amygdala suggests a disruption of effortful cognitive modulation of subcortical affect processing (pregenual ACC), as well as the development of negative self-referential emotional states (subgenual ACC).
A burgeoning literature has begun to illustrate the role of the striatum in aggression. Dysfunction of the ventral striatum likely contributes to aggression, owing to disturbances in the processing of expected outcome values, and therefore, phenomena such as “frustration.” Altered activity of the dorsomedial striatum may contribute to aggression by affecting the expected value or required effort associated with specific responses/actions.
Neurochemical systems are involved in aggression in at least two ways: (1) influencing central nervous system development during critical prenatal and postnatal periods, and (2) modulating developed neural system/network parameters. The relationship between 5-HT availability during development and aggression is complex. For example, the absence of 5-HT synthesis (tph2 knock-out) as well as excessive 5-HT synthesis capacity (high-activity tph2 polymorphism) can yield an aggressive phenotype in rodent genetic models. Furthermore, the prenatal and postnatal effects of 5-HTT pharmacological inhibition in animal models also appear to differ, as they lead to increased and decreased aggression, respectively. The developmental role of DA is also beginning to be elucidated, and excessive DA availability may contribute to a particularly severe form of aggression. A number of gene x environment interactions have been described, including polymorphisms of tph2, 5-HTT, MAOA, the D4 receptor and COMT, and early adversity.
Low basal levels of presynaptic 5-HT, particularly in corticolimbic regions, such as the ACC, are believed to contribute to trait levels of aggression, possibly by diminishing the efficacy of cognitive control of amygdala and striatal processes. Increased 5-HT2A receptor function in the medial OFC may influence state levels of aggression, possibly by decreasing OFC–amygdala coupling. The 5-HT1B receptor appears poised to influence aggression by its presynaptic location on 5-HTergic and GABAergic neurons projecting from the raphe and striatum, respectively, to the midbrain DAergic system. The 5-HT3 receptor also may play a role in aggression through a number of potential mechanisms, such as presynaptic modulation of DA release in the striatum. While clearly implicated in mood and anxiety disorders, the contribution of the 5-HT1A receptor in aggression is less clear; however, it may play a role in impulsivity.
Greater DAergic synthesis and storage capacity may attenuate aggression, possibly by modulating error/reward signals in striatal regions and/or by enhancing cortical cognitive processes. The neuropeptide VIP may enhance aggression through its actions in the central nucleus of the amygdala, in a manner possibly antagonistic to that of 5-HT’s effect. The steroid hormones cortisol and testosterone interact to determine aggression and related constructs, such as psychopathy, possibly through their effects on amygdala–frontal connectivity. The standard view has been that low cortisol permits high testosterone to promote aggression; however, their inter-relationship appears to be more complex and dependent on a number of other variables.
There are a number of essential questions that deserve the focus of future studies. The first relates to the various forms of heterogeneity and potential confounds in the study of aggression: What are the convergent and divergent neural correlates of aggression subtypes, namely, reactive and proactive aggression? Further, how do the neural correlates of reactive and proactive aggression relate to those of their associated personality dimensions, namely negative emotionality and impulsivity, and psychopathy, respectively? How does aggression differ between nonclinical participants, and personality disordered patients with and without IED? How do the various etiopathogenetic variants of aggression differ both phenomenologically and neurobiologically? And how do different etiopathogenetic factors interact – e.g., MAOA and 5-HTT risk polymorphisms, and do these interactions possibly contribute to qualitative differences in aggression severity? In addition to these phenomenological and etiopathogenic factors, it will also be essential that neuroanatomical regions are well defined with respect to their functional subdivisions.
Future neurobiological studies will also offer the opportunity to address important clinical goals. In this review, we described various candidate therapeutic targets that deserve further characterization, such as the 5-HT1B and 5-HT3 receptors, the D4 receptor, and the AVP V1a and V1b receptors. Therefore, PET imaging studies of these receptors may help to clarify their role in the pathophysiology of aggression, and pharmacological challenges with ligands to these targets, in combination with brain imaging and laboratory aggression paradigms, may provide initial evidence as to their anti-aggressive efficacy.
The genetic and developmental studies described here have laid the groundwork for identifying biomarkers that could be used to identify at-risk individuals and to develop potential interventions to disrupt the pathogenesis of aggression. Clarifying, for example, the differential contribution of 5-HT vs. DA in MAOA-dependent pathogenic mechanisms would allow for more rationally designed developmental interventions. Finally, as the neural circuitry of aggression is now better understood, dimensional biomarkers could be characterized that would represent sensitivity to specific treatments.
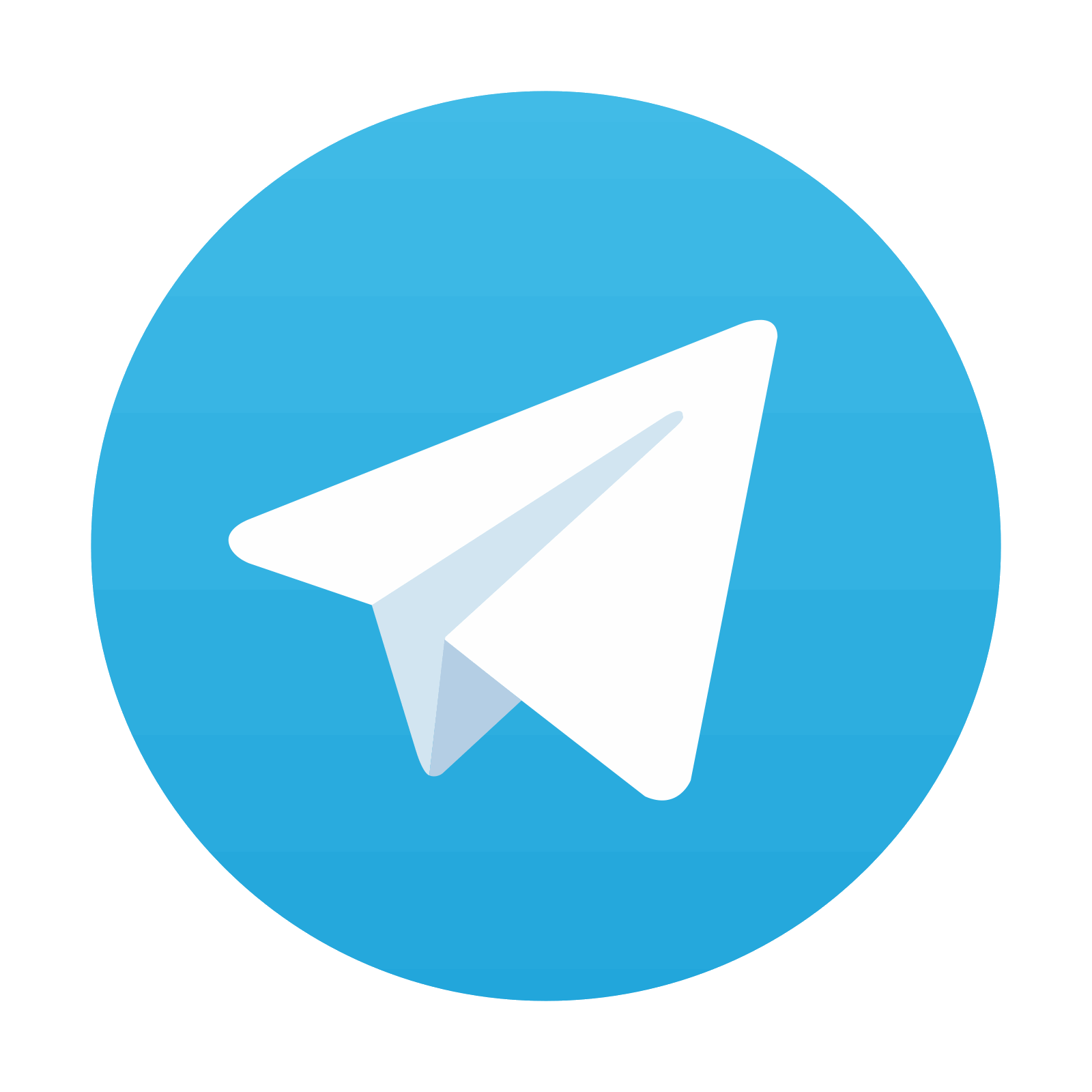
Stay updated, free articles. Join our Telegram channel
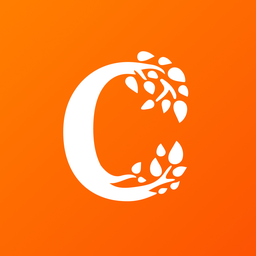
Full access? Get Clinical Tree
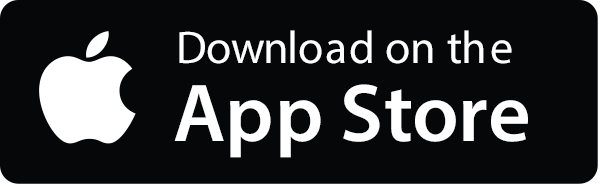
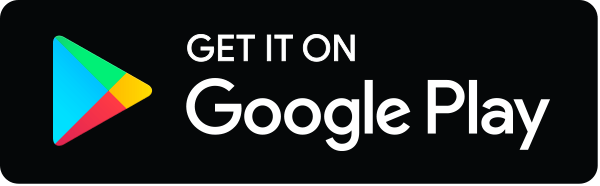