Chapter 17 The Visual System
It is clear from everyday experience that we are a visually oriented species. Although it is arguable which of our senses is most important, loss of vision certainly has a greater impact on humans than loss of, for example, olfaction or taste. Partly because of its importance (and partly for anatomical and technical reasons discussed later), a great deal of research has been done on the visual system. Currently, we know more about the visual system than about any other sensory system, and it is likely that with further study we will understand in some detail how this portion of the central nervous system (CNS) actually works.
The Eye Has Three Concentric Tissue Layers and a Lens
Eyes and cameras both need to deal with similar sets of issues—maintaining a stable relationship between a focusing apparatus and a photosensitive surface, focusing on near and far objects, regulating the amount of light reaching the photosensitive surface, and recording the pattern of incoming light—so, not surprisingly, they have many analogous components. The retina is an outgrowth of the diencephalon (Fig. 17-1), and one result of this origin is numerous parallels between the eye and the brain and meninges. The eye can be thought of as formed from three roughly spherical, concentric tissue layers, with a lens suspended inside them (Fig. 17-2). Each layer contributes to different structures in different parts of the eye (Table 17-1).
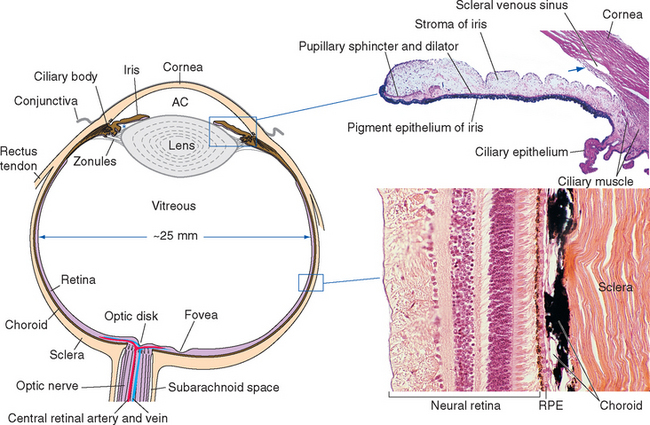
Figure 17-2 General structure of the eye as seen in a horizontal section, with histological sections of the iris and ciliary body (upper inset) and the wall of the eye (lower inset). The arrow indicates the trabecular meshwork overlying the scleral venous sinus (see Fig. 17-3). *, posterior chamber; AC, anterior chamber; RPE, retinal pigment epithelium.
(Upper inset, courtesy Caroline Steuer, University of Colorado Health Sciences Center. Lower inset, courtesy Dr. Allen L. Bell, University of New England College of Osteopathic Medicine.)
The heavily vascularized middle layer, the uvea* or uveal tract, is similar in some ways to the arachnoid and pia. This is the principal route through which blood vessels and nerves (other than the optic nerve) travel within the wall of the eye. Over most of its extent the uvea is sandwiched between the sclera and the retina as the densely pigmented choroid. Choroidal capillaries supply retinal photoreceptors, and choroidal pigment absorbs stray light (much like the flat black paint job inside a camera does). The uvea continues anteriorly to form the bulk of the ciliary body (containing the ciliary muscle) and the stroma of the iris.
The innermost layer is an outgrowth of the CNS and is itself a double-layered structure, reflecting its origin from the two layers of infolded optic cup (Fig. 17-1). Over most of its extent, this double layer comprises the retina, which lines the choroid. The outer portion of the retina, adjacent to the choroid, is the retinal pigment epithelium, whereas the inner portion, adjacent to the interior of the eye, is the neural retina. Under normal conditions no space exists between the pigment epithelium and the neural retina in adults. However, the mechanical connections between the two are not very strong, and under certain circumstances this potential space opens and retinal detachment results (see Fig. 17-14E). Retinal receptors are metabolically dependent on pigment epithelial cells and on the adjacent choroid-al vasculature, so detached areas stop working. The photosensitive retina ends anteriorly at a serrated border (the ora serrata), but the same two layers continue as the double-layered ciliary epithelium covering the ciliary body and the double layer of pigmented epithelium covering the posterior surface of the iris.
Intraocular Pressure Maintains the Shape of the Eye
Cameras have rigid bodies, designed to keep the film in a stable position relative to the lens. In contrast, the shape of the eye (and position of the retina) is maintained in much the same way as inflating a soccer ball maintains its shape. The collagenous sclera and cornea correspond to the wall of the soccer ball, and intraocular fluid pressure replaces air pressure. The pressure is generated by a now familiar process of fluid production, circulation, and reabsorption (Fig. 17-3). The ciliary body functions as a small outpost of choroid plexus, secreting aqueous humor across the ciliary epithelium and into the posterior chamber, the space between the iris and the lens. Pushed along by hydrostatic pressure, the aqueous humor passes through the pupil and into the anterior chamber, filters through the collagenous trabecular meshwork (analogous to arachnoid granulations) at the iridocorneal angle, and enters an endothelium-lined scleral venous sinus (the canal of Schlemm), which communicates directly with the venous drainage of the eye. The production rate (about 2 μL/min) is sufficient to completely replace the aqueous humor about 15 times a day. The space behind the lens, constituting most of the intraocular volume, is filled with gelatinous vitreous (Latin for “glassy”) humor, so the resistance to aqueous outflow afforded by the trabecular meshwork and the wall of the scleral venous sinus causes a pressure of about 15 mm Hg that is transmitted throughout the eye, maintaining its shape.
In much the same way that blocking the circulation or reabsorption of cerebrospinal fluid causes increased intracranial pressure, headache, and neural damage, processes that interfere with the circulation or reabsorption of aqueous humor cause the painful condition of glaucoma and, ultimately, retinal damage (see Fig. 17-14F and G).
The Cornea and Lens Focus Images on the Retina
Focusing an image requires refraction of light across one or more interfaces where there is a change in refractive index. The aqueous and vitreous humors have a refractive index only slightly lower than that of the lens, so the lens accounts for only about a third of the refractive power of the eye; its major role is adjusting the focus of the eye for near and far objects, as described later. Hence for nonaquatic vertebrates like us, most of the refraction occurs at the air-water interface at the front surface of the cornea;* its curved shape, maintained by intraocular pressure, accounts for our ability to see 90 degrees or more to the side (see Fig. 17-32).
One can imagine a variety of strategies for changing the focus of an optical device to accommodate to near objects—moving the photosensitive surface, moving the refractive elements, or changing the shape of the refractive elements. Different animals have adapted each of these strategies. Conventional cameras are adjusted for near or far objects by moving their lenses closer to or farther from the film; similarly, fish and most amphibians have intraocular muscles that move the lens back and forth. Arthropods cannot move or deform lenses that are part of the exoskeleton, but some have muscles that move the retina closer to or farther from the lens. Some animals have muscles attached to the cornea that can change its curvature. Terrestrial vertebrates use intraocular muscles to change the shape of the lens. Our lens is suspended by strands of connective tissue called zonules (Fig. 17-3, inset), attached at one end to the lens and at the other end to the ciliary body. At rest, the tension of this zonular suspension keeps the lens slightly flattened and the eye focused on distant objects. The ciliary muscle has some fibers oriented circumferentially that act as a kind of sphincter; contraction of these fibers pulls the ciliary attachment points of the zonules toward the center of the pupil and relaxes some of the tension in the zonular suspension. Other ciliary muscle fibers are oriented parallel to the surface of the eye; contraction of these pulls the ciliary attachment points partly anteriorly and partly toward the center of the pupil, again relaxing some of the tension in the zonular suspension. Hence, somewhat counterintuitively, contraction of the ciliary muscle allows the lens to fatten as the eye accommodates to near objects: the posterior surface of the lens is embedded in the vitreous humor and does not move much, but the anterior surface bulges out slightly.
The Iris Affects the Brightness and Quality of the Image Focused on the Retina
The size of the pupil is controlled by two smooth muscles in the iris (Fig. 17-2); both are highly unusual, in that they are derived from the same layers of neural ectoderm that give rise to the retina. The circumferentially arranged pupillary sphincter encircles the pupil at what was, embryologically, the edge of the optic cup.* The pupillary dilator, whose fibers are arranged like spokes radiating from the sphincter, is located at the interface between the pigment epithelial layers and the stroma. The sphincter is the stronger of the two, and reflex connections mediated by the optic and oculomotor nerves constrict the pupil in response to increased levels of illumination (see Fig. 17-39). The pupillary sphincter can contract by about 80%, much more than other muscles and enough to vary the diameter of the pupil from about 8 mm to 1.5 mm. However, this corresponds to only about a thirtyfold change in area, consistent with the idea that retinal mechanisms play the major role in adjusting visual sensitivity.†
In addition to decreasing the amount of light reaching the retina, a smaller pupil improves the optical performance of the eye (just as, within limits, a smaller aperture improves the optical performance of a camera lens). This is particularly important when focusing on near objects (see Figs. 17-40 and 17-41).
A System of Barriers Partially Separates the Retina from the Rest of the Body
A further indication of the origin of the retina from the neural tube is a blood-retina barrier system, parallel to the three-part barrier system in and around the brain (see Fig. 6-27), that separates the neural retina from other parts of the body. The endothelial cells of intraretinal capillaries, like those of intracerebral capillaries, are joined by bands of tight junctions, forming a blood-retina barrier in the literal sense of the term. Capillaries in the ciliary body are leaky, but the ciliary epithelium prevents diffusion into the aqueous and vitreous humors, just as the choroid epithelium prevents diffusion into cerebrospinal fluid. Finally, substances in the sclera and choroid are unable to reach the retina because retinal pigment epithelial cells are also joined by tight junctions, forming a layer analogous to the arachnoid barrier; traffic between choroidal capillaries and photoreceptors is mediated by transport across the pigment epithelium.
The Retina Contains Five Major Neuronal Cell Types
A simplified, schematic illustration of these basic connection patterns is shown in Figure 17-4. Starting peripherally, photoreceptor cells, stimulated by light, project to the first layer of synapses, where they terminate on the aptly named bipolar and horizontal cells. Bipolar cells then project to the next layer of synapses, whereas horizontal cells spread laterally and interconnect receptors, bipolar cells, and other horizontal cells. In the second layer of synapses, bipolar cells terminate on ganglion cells and amacrine cells.* Axons of ganglion cells leave the eye as the optic nerve, whereas processes of amacrine cells spread laterally and interconnect bipolar cells, ganglion cells, and other amacrine cells.
Retinal Neurons and Synapses Are Arranged in Layers
The entire retina is conventionally described as a 10-layered structure, beginning with the pigment epithelium (Fig. 17-5; see also Fig. 17-14); five of these layers are the layers of cell bodies and synapses just mentioned. In naming these layers, the term nuclear refers to cell bodies and the term plexiform to synaptic zones. Inner and outer refer to the number of synapses by which a structure is separated from the brain, so that, for example, photoreceptors are “outer” with respect to bipolar cells. From outside in, the 10 layers of the retina are as follows.
The outer segment of a rod is relatively long and cylindrical, whereas that of a cone (except in the fovea) is shorter and tapered (Figs. 17-6 and 17-7). Each type of outer segment is filled with hundreds of flattened membranous sacs, or disks. In cones, the interior of most of these disks is continuous with extracellular space, but in rods, almost all the disks have pinched off from the external membrane and are wholly intracellular. The major protein constituent of the outer segment membranes of both rods and cones is the visual pigment, which is called rhodopsin in rods. (There is no universally accepted name for the visual pigments of cones, and they are often referred to simply as cone pigments.) Hence photons traversing the outer segment of a rod or cone must pass through hundreds or thousands of sheets of membrane, each full of visual pigment molecules. As one might expect from this localization of visual pigment, the outer segment is the site of visual transduction; photons absorbed here cause a receptor potential that then spreads to the rest of the cell. The photosensitive portion of the receptor cells, oddly enough, is located in the part of the neural retina farthest removed from incoming light (i.e., the retina is inverted with respect to the path of light through it [Figs. 17-2 and 17-4]). This curious situation is universally true among vertebrates. However, this does not detract substantially from visual sensitivity or acuity, because the retina is thin (Fig. 17-5) and nearly transparent (Fig. 17-8), and because other anatomical modifications (discussed shortly) are found in the retinal area of greatest acuity.
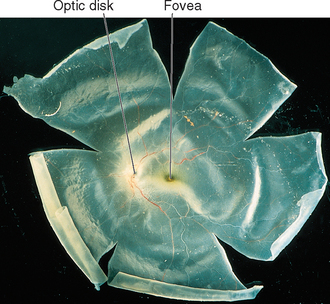
Figure 17-8 An isolated human neural retina.
(Courtesy Dr. Dennis M. Dacey, University of Washington School of Medicine.)
Each outer segment is an elaborately specialized cilium that remains connected to its inner segment by a narrow ciliary stalk. The inner segments contain, among other organelles, a very prominent collection of mitochondria. These mitochondria supply the energy necessary for processes associated with transduction and for the synthesis of visual pigments. These pigments are continually renewed, being synthesized in the inner segment, transported through the ciliary stalk, and incorporated into disk membranes. “Old” disks at the apical ends of the outer segments of rods and cones are then phagocytosed by the pigment epithelium. (Certain types of retinal degeneration are caused by a defect in this renewal-phagocytosis process.)
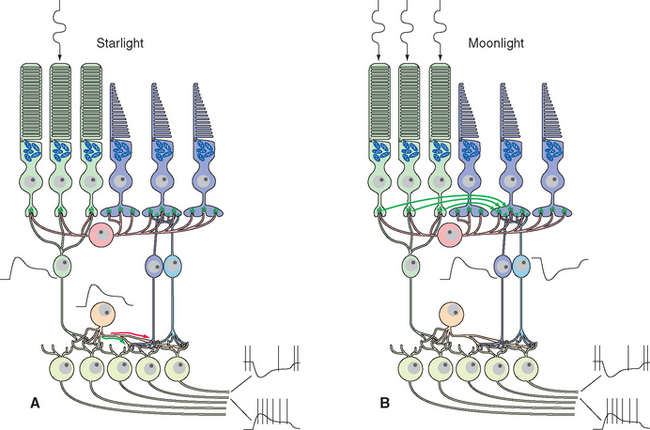
Figure 17-25 Rod signals reach ganglion cells through a remarkable system of special amacrine cells and modifiable gap junctions. At scotopic levels (A), rod signals reach rod bipolar cells, which hyperpolarize in response to glutamate (so they depolarize in response to light). Rod bipolar cells terminate on processes of special amacrine cells, in turn depolarizing them. Gap junctions open between amacrine cell processes and the synaptic terminals of cone ON-center bipolar cells (green arrow), allowing rod signals access to this branch of the cone circuitry. Other processes of the same amacrine cells make inhibitory (glycine) chemical synapses on the synaptic terminals of cone OFF-center bipolar cells (red arrow) and also on dendrites of OFF-center ganglion cells. At mesopic levels (B), gap junctions between rod and cone terminals open (green arrows), and rod receptor potentials gain access to the cone circuitry described in Figure 17-24.
The Retina Is Regionally Specialized
Cross sections through the retina do not have the same appearance at all locations. For example, no photoreceptors, interneurons, or ganglion cells are present at the optic disk, where the axons of ganglion cells leave the eye to form the optic nerve (Fig. 17-9). These axons originate near the vitreous, so they must turn posteriorly and traverse the retina before passing through the sclera. Because there are no photoreceptors at the optic disk, we are blind to any object whose image falls on this part of the retina. Although the blind spot can be demonstrated easily (Fig. 17-10), we have no awareness as we walk around a blank spot in visual space. One might think this is because the left eye can see the part of the visual field that falls on the right eye’s blind spot, and vice versa (see Fig. 17-32). This cannot be the explanation, though, because we are unaware of the blind spot even with one eye closed. The real reason is that the nervous system simply “fills it in.” We are actually quite skillful at this, and patients with damage to their visual systems can become blind in surprisingly large areas of their visual fields without being aware of it.
Beginning near the lateral edge of the optic disk is a circular portion of the retina, about 5 mm in diameter, in which many of the cells contain a blue-absorbing pigment. This gives the area a yellowish color (Fig. 17-8) when examined with appropriate illumination and has led to its being called the macula lutea (Latin for “yellow spot”), usually shortened to macula. In the center of the macula is a depression about 1.5 mm in diameter, called the fovea, which is particularly rich in cones. In the central part of the fovea is a pit, only about 350 μm across, that contains only elongated cones (no rods) and is directly in line with the visual axis (Fig. 17-11). The central fovea is specialized for vision of the highest acuity; all the neurons and capillaries that are present elsewhere (and that light would otherwise traverse before reaching the receptors) are collected around the edges of the fovea. Specialized interneurons called midget bipolar cells receive their inputs from individual foveal cones. These bipolars in turn contact individual midget ganglion cells, so that an anatomical basis for highly detailed foveal vision is maintained.*
The fovea is one extreme in a changing rod-cone distribution across the retina (Fig. 17-12). The packing density of cones decreases sharply outside the fovea, whereas that of rods increases, reaching a maximum just outside the macula. From here to the edge of the retina, the cone density remains at a low level, and the rod density slowly declines as well (Fig. 17-13). Given the properties of rods and cones, it follows from these distributions that the fovea is used for high-acuity color vision in reasonably bright light, whereas extrafoveal regions function at lower light levels.
< div class='tao-gold-member'>
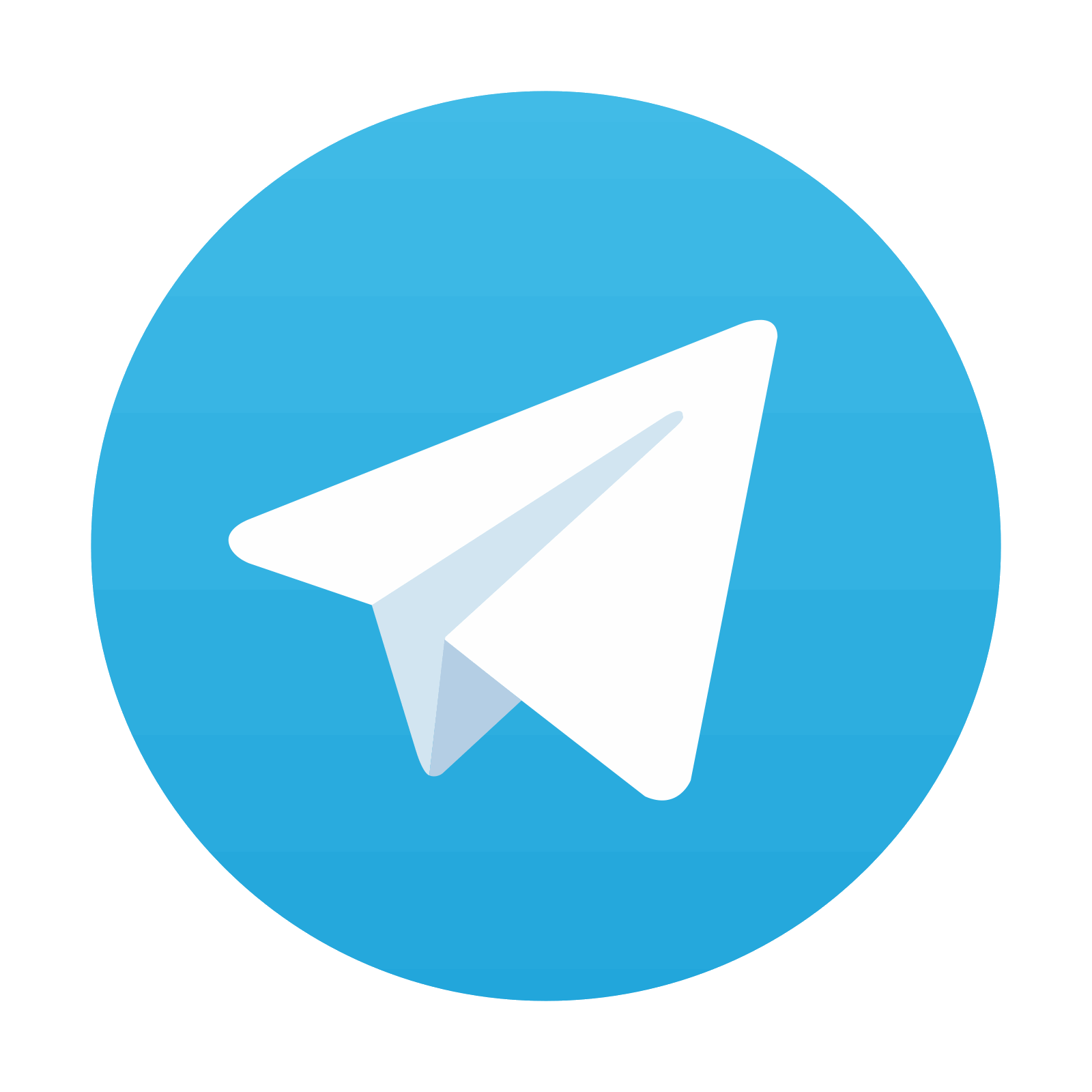
Stay updated, free articles. Join our Telegram channel
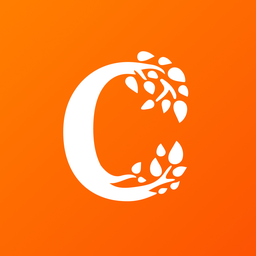
Full access? Get Clinical Tree
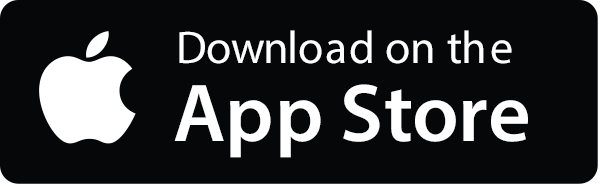
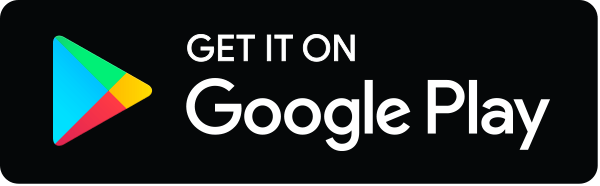