Introduction
Respiratory monitoring is an important aspect of managing patients admitted to the intensive care unit (ICU), including those with neurologic problems. Obviously, detecting gas exchange abnormalities is essential to properly manage many acute intracranial processes but neurologic patients often have coexisting pulmonary diseases and up to a third of patients admitted to a neurocritical care unit (NCCU) can develop acute lung injury (ALI) or acute respiratory distress syndrome (ARDS). Some possible causes for ARDS are listed in Table 20.1 . These may require complex ventilator management, which is facilitated by the availability of various types of pulmonary physiologic data. Although gas exchange monitoring is a well-established practice, the bedside measurement of respiratory mechanics is still relatively underused in the ICU. This stems from clinicians’ poor understanding of respiratory physiology, and because the evidence about the impact of this type of monitoring on outcomes is often confusing. This chapter provides a physiologic background on pulmonary function monitoring in the ICU, focusing on bedside measurement of gas exchange and respiratory mechanics, and highlights how these data can be used to support sound ventilator management choices. In addition how to decide who should be ventilated and criteria to determine liberation from mechanical ventilation is discussed briefly.
Direct Lung Injury | Indirect Lung Injury | |
---|---|---|
Common |
|
|
Less common |
|
|
Basic Respiratory Physiology and What It Means for Pulmonary Assessment
There are two compartments to the respiratory system: the lung and the chest wall. The chest wall includes the abdomen because abdominal pathology, including obesity or abdominal compartment syndrome can alter respiratory mechanics. To inflate the respiratory system requires pressure to be generated because of its resistive and elastic properties. Elasticity is expressed as compliance (volume change or pressure change). Esophageal pressure and airway pressure both should be measured to assess how each compartment of the respiratory system influences respiratory mechanics, particularly compliance. Most resistance comes from the airways, but small amounts are associated with tissue resistance, stress relaxation, and gas distribution.
Respiratory mechanics can be evaluated by several techniques. Among these the rapid airway occlusion technique that estimates alveolar elastic recoil pressure by measuring the inspiratory plateau airway pressure (Pplat) is a simple bedside test for ICU use. Intrinsic positive end-expiratory pressure (PEEPi) is another important variable that usually is measured using end-expiratory airway occlusion. PEEPi has important cardiopulmonary effects including decreased cardiac output, alveolar overdistention, increased work of breathing, and effects on compliance.
Imaging and Diagnostic Studies
A variety of imaging and diagnostic tools are available to evaluate pulmonary disease in the ICU, to identify high-risk populations, and as diagnostic tools to optimize mechanical ventilation. Basic tests include the bedside chest x-ray (CXR) and arterial blood gas analysis. Venous blood gas or evaluation of electrolytes and in particular serum bicarbonate may be useful for ventilator management when arterial blood gas analysis is not available. In patients who are difficult to wean, assessment of nutritional (e.g., phosphate) and endocrine status (e.g., cortisol and thyroid function) can be helpful. Noninvasive tests include computed tomography (CT), positron emission tomography (PET) or CT/PET, CT angiography for pulmonary embolus, electrical impedance tomography (EIT), and lung ultrasound (LUS). Invasive tests for diagnosis include bronchoscopy, bronchoalveolar lavage, transbronchial lung biopsy, and open lung biopsies. These invasive tests can be safely performed even in patients with ARDS. Common complications include transient hypoxemia, respiratory acidosis, and pneumothorax. Formal pulmonary function tests such as spirometry, diffusing capacity of the lung for carbon monoxide (DLCO), exercise testing, or bronchial challenges are infrequently performed on critical care patients. In the future biomarkers and genomic analysis may become useful tools in diagnosis or identification of patients at risk for pulmonary disorders such as ALI or ARDS.
The bedside CXR can reveal abnormalities that may not be clinically evident. In addition, a CXR can detect the malposition of tubes, including an endotracheal tube and catheters and identify complications associated with these devices. Today quantitative CT is the gold standard to assess lung morphology, recruitment, and hyperinflation of lung tissue at different inflation pressures. LUS is a useful, radiation-free, noninvasive bedside lung-imaging tool. Like CT it is primarily a tool to detect changes in density. EIT and PET provide more functional data. For example, EIT can provide a noninvasive continuous image of pulmonary impedance. This can be useful during mechanical ventilation because it indicates the distribution of ventilation. PET also can be used to assess lung inflammation that along with impaired gas exchange is observed in ARDS, ALI, or ventilator-induced lung injury (VILI).
ARDS and ALI
Many patients in the ICU can develop ALI or ARDS, and patients with severe acute brain injury often are at risk for developing these conditions. Mortality from ALI and ARDS remains high and their development adversely affects intracranial physiology. It is important then to know how to define these conditions, recognize who is at risk for them, and diagnose their onset ( Tables 20.1 , 20.2 , 20.3 , and 20.4 ). ARDS is a clinical-radiologic diagnosis and includes severe hypoxemia assessed by an arterial oxygen tension/fraction of inspired oxygen ratio less than 200 and bilateral infiltrates on a CXR in the absence of left atrial hypertension. The definition of ARDS and ALI is provided in Table 20.2 . Other sets of definition criteria have been proposed (see Tables 20.3 and 20.4 ). In children ALI and ARDS often are defined by the oxygenation index defined as the product of mean airway pressure (MAP) × FiO 2 × 100/PaO 2 . However, the sensitivity and specificity of the clinical diagnosis may depend on the underlying etiology. Other diagnostic tests are used in large part to exclude conditions that may mimic ALI or ARDS or to guide therapy. Chest CT can help evaluate the extent of the disease and guide ventilation and prognosis. Current therapy is centered on lung-protective mechanical ventilation and a restrictive fluid management strategy.
ALI criteria |
Timing: acute onset Oxygenation: PaO 2 /FiO 2 ≤300 mm Hg (regardless of PEEP level) Chest radiograph: bilateral infiltrates on frontal chest radiograph Pulmonary artery wedge pressure: ≤18 mm Hg when measured or no clinical evidence of left atrial hypertension |
ARDS criteria |
Same as ALI except oxygenation: PaO 2 /FiO 2 200 mm Hg (regardless of PEEP level) |
Variable | Score |
---|---|
1. Chest roentgenogram score | |
No alveolar consolidation | 0 |
Alveolar consolidation confined to 1 quadrant | 1 |
Alveolar consolidation confined to 2 quadrants | 2 |
Alveolar consolidation confined to 3 quadrants | 3 |
Alveolar consolidation in all 4 quadrants | 4 |
2. Hypoxemia score | |
PaO 2 /FiO 2 >300 | 0 |
PaO 2 /FiO 2 225-299 | 1 |
PaO 2 /FiO 2 175-224 | 2 |
PaO 2 /FiO 2 100-174 | 3 |
PaO 2 /FiO 2 <100 | 4 |
3. PEEP score (when ventilated) (cm H 2 O) | |
PEEP ≤5 | 0 |
PEEP 6-8 | 1 |
PEEP 9-11 | 2 |
PEEP 12-14 | 3 |
PEEP >15 | 4 |
4. Respiratory system compliance score (when available) (mL/cm H 2 O) | |
Compliance >80 | 0 |
Compliance 60-79 | 1 |
Compliance 40-59 | 2 |
Compliance 20-39 | 3 |
Compliance <19 | 4 |
* The final score is calculated by the addition of the component parts: score 0, no lung injury; score 1 to 2.5, mild to moderate lung injury; score >2.5, severe lung injury.
Definition | Pros | Cons |
---|---|---|
AECC |
|
|
Murray score |
|
|
Delphi |
|
|
Oxygenation index |
|
|
Gas Exchange Monitoring
History
Blood gas analysis was introduced by Severinghaus in 1958 and rapidly became an essential part of the clinical routine. This technique provides direct measurement of arterial blood PO 2 , PaCO 2 , and pH and also allows for calculation of serum bicarbonate, base deficit-excess, and oxyhemoglobin saturation. The last can be directly measured using co-oximetry, which also measures carboxyhemoglobin and methemoglobin.
The history of pulse oximetry started in the 1930s, with the first studies on the absorption of light by oxygenated blood. However, the first clinically acceptable instruments became available only in the 1970s. Their introduction made bedside monitoring of arterial oxygenation possible in the ICU, in the operating room (OR), and in many other hospital locations. Capnometry, the measurement of carbon dioxide (CO 2 ) in the expired gas, was first made possible by infrared photometry in the 1940s. A subsequent step was the introduction of capnography, which is the analysis of expired CO 2 versus time waveforms. This advancement followed the realization that these tracings have profound physiologic meanings and that their analysis provides useful clinical information in ventilated patients. A more recent addition is the development of volumetric capnography, which is the analysis of CO 2 over volume waveforms and provides further pathophysiologic insight. Similar to pulse oximetry, capnometry and capnography have become part of the clinical routine in the OR, although their use is still somewhat limited in ICU patients.
Engineering and Technology
Pulse oximeters determine oxygen saturation (SpO 2 ) using the spectrophotometric characteristics of pulsatile arterial blood. Oxyhemoglobin absorbs light in the infrared spectrum at 940 nm, whereas deoxyhemoglobin absorbs light preferentially at 660 nm, corresponding to red light. The pulse oximeter probe consists of two diodes, each emitting red or infrared light into tissue, and of sensors that measure the emerging amount of radiation. SpO 2 is obtained by processing the relative absorption of light by oxygenated and nonoxygenated hemoglobin. The device reports only the pulsatile component of the recorded signal, and the final result is a continuous measurement of the patient’s oxyhemoglobin status and pulse rate. Calibration is done in volunteers who breathe hypoxic mixtures of gas. Standard, bi-wavelength pulse oximetry does not detect the presence of dyshemoglobinemias, but multiwave pulse oximeters recently have been developed. These instruments also can estimate total hemoglobin concentration together with carboxyhemoglobin and methemoglobin levels.
Clinical capnometry is performed using a source of infrared light and measuring the absorption by CO 2 at various wavelengths, using a photodetector within a sampling cell. The measured light absorption is then compared with a CO 2 -free reference, and finally CO 2 is expressed either as partial pressure or as concentration. There are two types of capnometers: (1) mainstream capnometers measure CO 2 directly in a cuvette placed in the ventilator circuit, close to the endotracheal tube, and (2) sidestream capnometers aspirate gas from the airway opening using a small-bore tubing line; CO 2 is then measured in a chamber placed within the monitor. Thus CO 2 measurements lag behind the respiratory cycle in sidestream capnometry. All modern capnometers also provide a capnography tracing on the display screen. Volumetric capnographs combine a mainstream capnometer with a flowmeter within the same device, thus providing measurements of CO 2 production and dead space. In this technique, flow and CO 2 signals need to be synchronized for measurements to be meaningful.
Indications for Use
Pulse oximetry is currently part of the basic ICU monitoring and is applied to almost all patients irrespective of their clinical status: in fact SpO 2 is often referred to as the “fifth vital sign.” The main purpose of SpO 2 monitoring is to provide an early warning of hypoxemia and to avoid its complications. In ventilated patients, SpO 2 also is used to titrate oxygen therapy and positive end-expiratory pressure (PEEP). This decreases the need for frequent arterial blood sampling. Capnometry and capnography are considered a standard in the OR, where they are mainly used to assess the adequacy of the airway and of ventilation. In particular, capnometry is the most effective method to verify successful intubation. In the ICU, capnometry is mostly used for select patient populations, such as those with intracranial hypertension, where it provides a surrogate for continuous monitoring of arterial PaCO 2 , the control of which can be important in management of increased intracranial pressure (ICP). Capnometry also can be used to facilitate ventilator weaning and at the same time limit blood sampling. However, there are limitations of this monitor as an estimate of arterial PaCO 2 that should not be disregarded in patients with lung diseases (see following text).
Information Provided and Troubleshooting
Pulse oximetry is used to measure arterial saturation noninvasively and accurately predicts arterial PO 2 . However, the oxyhemoglobin dissociation curve has a sigmoid shape, and on its flat portion large PO 2 decreases may cause only minimal changes in SpO 2 and be underestimated. Furthermore, the accuracy and precision of pulse oximetry measurements decrease when hemoglobin saturation is less than 90%. For these two reasons, any decrease in the SpO 2 below 90% must be considered a significant clinical event and assumed to predict hypoxemia. Saturations of less than 80% do not convey any meaningful information other than that the patient is severely hypoxemic, because no calibration data on human subjects are available in this range. The use of standard pulse oximeters is limited in the presence of dyshemoglobinemias, and thus co-oximetry should be performed whenever carbon monoxide poisoning is suspected. Hypotension, vasoconstriction, and hypothermia reduce the pulsatility of capillary blood and the ability of the pulse oximeter to identify a tracing. In addition, dark skin pigmentation decreases the accuracy of SpO 2 measurement. This same study also suggested that a higher SpO 2 goal should be used when titrating oxygen therapy in black or dark-skinned rather than fair-skinned patients.
Capnography tracings are characterized by stages that correspond to specific moments of the respiratory cycle ( Fig. 20.1, A ). At the start of exhalation, measured PaCO 2 is negligible because the airways contain only fresh gas (point 1). The volume of the airways is called anatomic dead space because the gas they contain does not participate in gas exchange. As expiration proceeds, CO 2 -rich alveolar gas enters the airways, causing a rapid increase in PaCO 2 (points 2 to 3). When the dead space has been completely washed out by alveolar gas, the exhaled PaCO 2 curve reaches a plateau (points 3 to 4). During inspiration, fresh gas enters the airways and PaCO 2 rapidly drops to zero again (point 5). The highest measured PaCO 2 , identified at the end of expiration (point 4) is called end-tidal CO 2 (etCO 2 ) and is indicative of the PaCO 2 in the alveoli. Because the alveolar gas is in equilibrium with the pulmonary capillary blood, etCO 2 is also very close to arterial PaCO 2 in healthy subjects and it can be used to adjust ventilation. However, etCO 2 may underestimate arterial PaCO 2 in the presence of diseases that cause significant alveolar dead space, such as chronic obstructive pulmonary disease (COPD), pulmonary embolism, and ARDS. Alveolar dead space is the wasted fraction of inspired gas that does not participate in CO 2 exchange because of the presence of alveolar units with low or absent perfusion relative to ventilation. In this case, gas exhaled by such units contains very low or negligible CO 2 , thus causing etCO 2 to be lower than the arterial PaCO 2 ( Fig. 20.1, B ). The difference between the two variables is proportional to the fraction of alveolar dead space. Thus in patients with lung disease, etCO 2 should never be assumed to accurately represent arterial PaCO 2 unless an association between the two variables can be confirmed by arterial blood gas analysis. When there is a discrepancy, the trend in etCO 2 still can be used. Volumetric capnography allows assessment of both anatomic and alveolar dead space, together with CO 2 production. These variables can be used to titrate mechanical ventilation, to detect changes in hemodynamic status and pulmonary embolism, and to confirm diagnoses and prognoses.

Analysis of capnographic tracings provides further information, and the clinician should be aware of a number of abnormal patterns. In patients with COPD and asthma, the upstroke of expired CO 2 is slower than normal and a plateau stage is never reached. In this case, etCO 2 and arterial PaCO 2 cannot be determined. This phenomenon results from severe dishomogeneity of expiration kinetics among different alveolar units. An exponential decrease in etCO 2 over minutes is an ominous sign, because it indicates a rapid decrease in pulmonary perfusion, which can be due to pulmonary embolism, sudden onset of shock, or impending cardiopulmonary arrest. Capnography also can be used to detect leaks in the breathing circuit, disconnection from the ventilator, and a patient’s inspiratory attempts.
Therapy Based on Gas Exchange Monitoring and Its Effect on Outcome
Both pulse oximetry and capnometry became widely accepted for clinical use in the mid- to late 1980s. Since then, multiple medical societies have recommended the use of these monitors, particularly in the OR but also in other settings. It is very likely that the introduction of SpO 2 and capnometry has contributed to improved perioperative morbidity and mortality. This statement is based mostly on an analysis of closed anesthetic malpractice claims that suggested that a majority of intraoperative respiratory mishaps would have been avoided had pulse oximetry and capnometry been available. The same dataset also showed a historic trend of reduction in respiratory-related claims following introduction of these monitors. However, the strength of clinical evidence that supports the use of capnometry and pulse oximetry in the perioperative setting and in the ICU is disappointing, although there is evidence to suggest use of pulse oximetry makes a difference to patient care. First, randomized studies conducted in the perioperative period show that the use of pulse oximetry increases the rate of detection of hypoxemia. Second, Moller et al. in a randomized study that included 28,802 patients observed that patients who received SpO 2 monitoring had a lower incidence of intraoperative electrocardiographic ischemic changes than control patients who did not have a SpO 2 monitor. However, the overall rate of perioperative complications and the mortality were similar in the two groups. In a smaller study from the same group, the rate of cognitive dysfunction was similar in patients who were or were not monitored with pulse oximetry. A 2003 Cochrane review concluded that although pulse oximetry improved detection of hypoxemia, its utility and cost effectiveness as a tool to improve outcomes is questionable, at least in the perioperative period. This observation was confirmed in a subsequent Cochrane analysis of five randomized trials that included 22,992 patients and compared pulse oximetry or no pulse oximetry during the perioperative period (i.e., there was no difference in mortality although the incidence of hypoxemia in the recovery room was less in the pulse oximeter group). However, given the wide acceptance of this monitoring technique, it is unlikely that further randomized studies will be performed in the future.
Capnometry, and particularly volumetric capnography provides helpful physiologic information that can be used to guide mechanical ventilation and weaning. Dead space is also an independent predictor of mortality in ARDS patients. However, there is a lack of outcome evidence that this form of monitoring is helpful in the ICU, other than for the confirmation of intubation.
Risks
Pulse oximetry and capnometry are essentially risk-free techniques, from the point of view of device safety. Similar to any other type of monitoring, it can be argued that patient harm may result from poor interpretation of the data and lack of understanding of the technique, rather than from the monitor itself. An example of a common misunderstanding is the assumption that a good SpO 2 value also reflects adequate ventilation. In reality, oxygen therapy in a hypoventilated patient may effectively correct hypoxemia while masking severe hypercapnia, thus giving clinicians a false sense of security. This phenomenon could be the cause of adverse respiratory events during procedural sedation and can be prevented if capnometry is performed with pulse oximetry.
Respiratory Mechanics and Ventilator Variables
History
The conceptual background to respiratory mechanics monitoring is provided by classic pulmonary physiology. Studies on pressure-volume loops obtained during inflation and deflation of surfactant deficient lungs showed characteristic abnormalities that later in time also were observed in patients with ARDS. However, it was the introduction of the rapid flow interruption technique that brought the measurement of respiratory system compliance and resistance to the bedside. Respiratory mechanics have become more relevant in critical care since the demonstration that excessive alveolar inflation leads to ventilator-associated lung injury (VALI) and can worsen patient outcomes.
The use of esophageal pressure (Pes) to measure work of breathing and to separately study the mechanics of the lung and of the chest wall was initially tested in studies on healthy volunteers and in patients with COPD. In the 1990s, this technique was extended to ICU patients and led to an understanding of the importance of chest wall mechanics and patient-ventilator dyssynchrony (PVD) that can increase the work of breathing. Impulse oscillometry can be used as a noninvasive method to assess pulmonary compliance rather than using the esophageal pressure method, which is invasive.
The advent of CT of the chest in ARDS patients led to the demonstration that ventilation is not homogeneous and that atelectasis is relevant in this syndrome. In particular, CT is able to reveal discrepancies between bedside chest x-rays and various clinical and physiologic parameters in ARDS patients. For a long time, PEEP was used to achieve goal oxygenation levels through alveolar recruitment in ARDS and in other forms of acute respiratory failure. The discovery of cyclic alveolar collapse and reopening in human ARDS, and animal evidence that these phenomena contribute to VALI led to the formulation of the “open lung concept.” According to this idea, optimization of alveolar recruitment may improve patient outcomes and should be a goal of ventilator management, independent from the oxygenation response. Although the results of randomized studies on this topic have been contradictory, the open lung concept has renewed the interest in bedside clinical tools to measure alveolar recruitment, lung volumes, and regional patterns of ventilation.
Engineering and Technology
Monitoring respiratory mechanics requires pneumotachographs and electromechanical transducers to measure gas flows and airway pressures (Paw). Pneumotachographs quantify flow from the pressure drop generated by the passage of gas through a known resistance, which typically includes a thin metal grid to maintain laminar gas flow. Other designs are available, although this is the most frequently used flowmeter. A differential pressure transducer converts the pressure difference across the resistance into an electrical signal, which then is amplified, calibrated, and processed. Volumes are obtained by mathematical integration of the measured flow. Transducers typically are embedded in the ventilator machinery, remotely from the patient and serve the dual purpose of feeding the ventilator servocontrols and of providing data for display. Modern ventilators also display curves, facilitating detection and interpretation of events. In certain ventilators, flows and pressures are measured at the proximal opening of the endotracheal tube, bypassing the ventilator tubing. This design renders the measurements more accurate but exposes the transducers to vapor condensation and mucus accumulation.
Many ventilators allow the clinician to perform a rapid flow interruption maneuver at the end of inspiration or expiration by pushing a dedicated button on the ventilator console. During this maneuver both the inspiratory and the expiratory valves of the ventilator remain closed for a short time and so provide conditions of zero flow. This maneuver is fundamental to the measurement of respiratory mechanics variables at the bedside. An algorithm that automatically performs repetitive, short-flow interruptions and provides frequently updated measurements is available, but it has been created specifically to be implemented in the proportional assist ventilation (PAV+) mode.
Pes is measured using a thin, 10-cm-long balloon partially filled with air and inserted into the lower third of the esophagus. The use of central venous pressure as a surrogate for Pes also has been described. Pes measurement is included in pulmonary monitoring systems provided with disposable esophageal catheters and pneumotachographs.
Pressure volume loops are obtained by step-wise or continuous inflations-deflation cycles of the lungs, which are performed using either a large-volume syringe or a ventilator with dedicated software. Graphic or mathematical analysis is used to extrapolate clinically useful information from these loops.
EIT is a relatively new bedside imaging technique that constructs an image of gas distribution within the chest during the different phases of respiration using a series of electrode pairs placed around the circumference of the chest Potential applications for this technique include titration of PEEP, recruitment maneuver, and assessment of regional patterns of ventilation. Although commercial devices are available, their clinical application has still to be fully elucidated. Techniques that measure lung volumes through gas dilution or washout techniques are in the process of being tested and may be used in the future to help optimize ventilator parameters.
Indications for Use
Monitoring of Paw, tidal volume (TV), expired minute volume (VE), and other basic ventilator variables is essential to the care of all ventilated patients. These data document the effectiveness of ventilation, they signal disconnections from the ventilator and airway obstruction, and they provide early warnings of changes in patient condition. In many institutions these are the only variables that are collected during routine patient management and are probably sufficient in the majority of patients. However, slightly more advanced monitoring is easy to perform and can be very useful in many cases. First, visual inspection and interpretation of Paw and flow versus time tracings can help detect asynchronies between patient and ventilator ( Fig. 20.2 ). Furthermore, the flow interruption method should be used periodically in every ventilated patient if the machinery allows it. This technique is associated with no additional risk or cost and allows the measurement of compliance, inspiratory resistance, and intrinsic PEEP (PEEPi), all variables that can rapidly reveal changes in patient conditions.
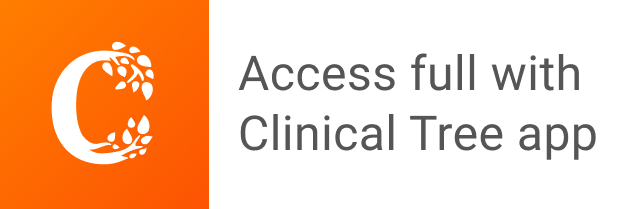