Introduction
The injured brain is exquisitely sensitive to global changes in blood flow and oxygen delivery. Brain injury rarely occurs in the absence of systemic injury, either as a primary (trauma) or secondary process (subarachnoid hemorrhage). Hence, volume and circulatory abnormalities may induce or worsen brain injuries, and brain injuries may induce circulatory abnormalities. In the setting of cerebral autoregulatory dysfunction, large swings in cardiac output or peripheral resistance may have an adverse effect on brain tissue perfusion. Furthermore, manipulation of volume status and blood pressure is central to the management of conditions such as subarachnoid hemorrhage (SAH) and traumatic brain injury (TBI) to maintain cerebral perfusion pressure (CPP) or prevent delayed cerebral ischemia (DCI). Whereas neurocritical care focuses on neurologic monitoring, cardiovascular monitoring is performed on all neurologically injured patients and the data derived are central to all therapeutic decisions. However, there is no single set of optimal hemodynamic or cardiac values that applies to all patients. For example, an adequate blood pressure to avoid cerebral hypoperfusion likely is different in a young individual with no preexisting disease compared with an older patient who has hypertension and atherosclerotic disease. Consequently, an understanding of the methods of cardiovascular monitoring, the principles behind them, and interpretation of hemodynamic data is a core competence for the neurointensivist. This chapter discusses techniques to monitor volume status and cardiac function. The evaluation of cardiac ischemia, acute coronary syndromes, and use of biomarkers in these disorders is beyond the scope of this chapter, and the reader is referred to recent reviews on these topics.
Physical Examination and Laboratory Determination of Effective Circulating Volume
Evaluation of volume status, preload, and fluid responsiveness is important in neurocritical care because hypotension is a well-known secondary cerebral insult. In addition, volume expansion or vasopressors frequently are used to augment cerebral blood flow (CBF). However, positive fluid balance or induced hypertension also can have adverse effects on outcome or intracranial physiology. Hence, an understanding of a patient’s volume status is fundamental to his or her care. Acute and chronic intravascular fluid deficits may manifest with varying degrees of clinical findings. Patients with chronic volume deficits may present with a decreased skin turgor, weight loss, sunken eyes, hypothermia, oliguria, orthostatic hypotension, and chronic low-grade tachycardia. On the other hand, those with acute volume deficits may present clinically with changes in vital signs, with tachycardia and hypotension, oliguria, and anuria, but also with more subtle changes in mental status, such as restlessness and confusion. Clinical signs of volume excess include weight gain, pulmonary congestion or edema, and peripheral edema.
Basic laboratory tests should be obtained to help determine effective circulating volume. An increase in all plasma electrolyte concentrations may indicate volume loss, but creatinine and blood urea nitrogen (BUN) elevations may be more specific. In particular, a BUN-to-creatinine ratio greater than 15 may be more sensitive to indicate an intravascular fluid deficit. In systemic volume loss, renal sodium retention results in urinary sodium concentration (UNa) that typically drops below 20 mEq/L. The fractional excretion of sodium (FENa) is considered more sensitive and can be calculated by the formula
UNa × PCr / PNa × UCr × 100 %
where U and P are urine and plasma measured concentrations of sodium and creatinine. A FENa less than 1% indicates a reduced effective circulating volume seen by the kidney provided no diuretics have been administered in the previous 24 hours.
Although these clinical and laboratory evaluations usually are adequate to monitor the surgical or medical patient in the outpatient or ward setting, they may often be insufficient and inconclusive in the critically ill patient. Instead, more sophisticated, continuous and real-time methods of hemodynamic cardiovascular monitoring become necessary to help determine adequate global tissue perfusion.
Blood Pressure Monitoring
Blood pressure, universally measured in intensive care units (ICUs), reflects myriad factors that maintain the systemic circulation. These include, but are not limited to, circulating blood volume (both stressed and unstressed) venous return, splanchnic venular and arteriolar tone, vascular resistance within several microcirculations, heart rate, cardiac compliance, right ventricular afterload, airway pressure, left ventricular contractility, core body temperature, baroreceptor reflexes and adrenal medullary output. Distilling these factors into a single figure—the mean arterial pressure—to guide therapy is fraught with hazard. In simple terms, blood pressure reflects stroke volume and peripheral resistance. However, compensatory reflexes in one of these parameters may balance variability in the other. An example is vasoconstriction in the setting of hypovolemia. The result may be misleadingly “normal” blood pressure despite severe systemic disease. Conventionally, blood pressure is measured two ways: noninvasively, using manual techniques or oscillometry, and invasively, using direct arterial cannulation.
Noninvasive
Manual Intermittent Techniques
To measure both systolic and diastolic arterial pressure, the most widely used intermittent manual method is the auscultatory technique, originally described by Scipione et al. but popularized by Korotkoff in 1905. Using a sphygmomanometer, cuff, and stethoscope, Korotkoff measured blood pressure by auscultation of the sounds generated by arterial blood flow. These sounds are a complex series of audible frequencies produced by turbulent flow, instability of the arterial wall, and shock wave formation as external occluding pressure on the artery is reduced. The pressure at which the first Korotkoff sound is heard generally is accepted as systolic pressure (phase I). The sound character progressively changes (phases II and III), becomes muffled (phase IV), and is finally absent (phase V). Diastolic pressure is recorded at phase IV or V. However, phase V may never occur in certain pathophysiologic states such as aortic regurgitation. In 1905, Harvey Cushing recommended the routine use of the noninvasive mercury sphygmomanometer in intraoperative management of patients.
The sphygmomanometer method has several shortcomings. In particular, it is important that measurements are consistent from observer to observer in critical care. However, if the cuff is released too rapidly or if the observer fails to identify sounds, then incorrect measurements may be recorded. The sounds may be particularly difficult to hear in shock states or with tachyarrhythmias, making inherent interobserver variability relevant in this situation. Moreover, if the patient is shivering or edematous, the blood pressure may appear higher than normal. A slight bend in the limb on which the cuff is placed also may lead to falsely elevated recordings, whereas leaks in the pneumatic system may lead to falsely lower recordings. Finally, cuff size and fit are also of critical importance. The width of the cuff should be 20% greater than arm diameter. If the cuff is too narrow or too short the blood pressure will be overestimated. Many of these problems also are associated with continuous blood pressure measurement using oscillometry.
Oscillometry
Many limitations of manual intermittent blood pressure measurement have been overcome by automated noninvasive blood pressure (NIBP) devices. NIBP devices measure mean arterial pressure and use an algorithm to estimate the systolic and diastolic blood pressure. The major advantages of automated blood pressure monitoring are reliability, reproducibility, and built-in low- and high-pressure alarms. In addition, in modern devices data are transferred directly into the monitor or information system, freeing the bedside caregiver for other duties. This may result in more frequent sampling but perhaps less attentiveness to the results.
Most automated NIBP devices are based on oscillometry. This monitoring system consists of pneumatic and electronic components. Modern oscillometers use a single cuff to occlude the artery and sense the arterial signal. The cuff is inflated above systolic pressure and then deflates either continuously or in a step-wise manner. As the cuff pressure decreases, at a rate about 4 mm Hg per second, below occlusive pressure, blood starts flowing through the artery and causes detectable oscillation. The pulse pressure wave and the gauge pressure in the occluding cuff are detected and converted into an electronic signal by a transducer. The pressure at which the peak amplitude of arterial pulsations occurs corresponds closely to directly measured mean arterial pressure (MAP), and values for systolic and diastolic pressure are derived from proprietary formulas that examine the rate of change of the pressure pulsations. A microprocessor uses an algorithm to determine systolic, diastolic, and mean arterial pressures. Systolic pressure is identified as the pressure at which pulsations are increasing and are at 25% to 50% of maximum. Diastolic pressure is the most difficult value to determine by oscillometry and is commonly recorded when the pulse amplitude has declined from the peak value by 80%. Oscillometers are generally safe and reliable; however, similar to manual intermittent techniques, care must be taken to ensure proper cuff size.
Invasive
Continuous, invasive blood pressure is the gold standard used to monitor blood pressure in critically ill patients. First described 60 years ago, it is now considered the most reliable method, especially in hypotensive and vasoconstricted patients. The beat-to-beat and continuity of measurement allow early diagnosis and intervention.
This technique requires an intra-arterial cannula, fluid-filled tubing, a pressure transducer and a microprocessor with a display. The pressure transducer is the key component of the system. It is a low-compliance diaphragm that moves in response to pressure changes transmitted through fluid filled tubing. This movement is converted to electrical signals, most commonly using a strain gauge set-up, based on the principle that electrical resistance of a wire changes with changes of its length. There are four wires or strain gauges that form a electrical circuit based on the Wheatstone bridge. In this set-up, with any movement of the diaphragm two wires are compressed and the two other stretched, thus increasing the sensitivity to diaphragm movement four times. Modern technology uses semiconductors or silicone crystals instead of wires. The MAP is calculated by integrating the area under the waveform, and the systolic and diastolic pressures calculated using an algorithm. The arterial pressure waveform is made of multiple sine waveforms with different frequencies that can potentially match the natural oscillatory frequency of the system and resonance and distort the signal. The monitoring system is designed to keep its frequency above the arterial waveform frequencies for accurate measurement.
The major problems associated with invasive blood pressure monitoring are dampening and resonance; these can affect accuracy of the blood pressure waveform and measurement. Dampening decreases the rate of signal change and so leads to low pulse pressure with low systolic and high diastolic pressure readings. Arterial cannula occlusion, air bubbles, and soft tubing are common causes of an over-dampened signal. Interestingly, MAP accuracy is preserved because of energy conservation; hence, if the waveform is dampened, the mean pressure is accurate but the systolic and diastolic are not.
Strengths, Limitations, and Clinical Indications for Noninvasive and Invasive Blood Pressure Monitors
Both invasive and noninvasive blood pressure monitors may damage local tissue. Although noninvasive automated blood pressure measurement techniques are considered relatively safe, repetitive cuff inflation may cause patient discomfort and persistent arterial occlusion that may result in pain, petechiae and ecchymoses, limb edema, venous stasis and thrombophlebitis, peripheral neuropathy, and rarely compartment syndrome ( Table 19.1 ). These events occur more often after prolonged periods of excessively frequent cuff inflation-deflation cycling and result from local trauma. Other factors that may contribute to complications include cuff misplacement across a joint, or repeated attempts to determine blood pressure in the presence of an artifact-producing condition such as involuntary muscle tremors.
|
Most invasive arterial lines are placed in the radial artery because of easy access and protective collateral flow by the ulnar artery. This makes digital ischemia an uncommon complication. Although other arterial sites are described, the femoral artery is the most common alternative, preferred by some over the radial site because it may better reflect central pressures and its luminal pressure is perhaps less affected by peripheral vascular disease or arteriosclerosis. The brachial and axillary arteries also may be used but have less collateral circulation to counteract end limb ischemia. The major complication of arterial lines is local thrombosis. In the majority of arterial lines some degree of nonocclusive thrombosis occurs without flow compromise. This is seen in more than 50% of radial lines, with up to 15% resulting in complete occlusion. The main risk factors for occlusive thrombosis are catheter size relative to vessel size, the number of arterial punctures needed to insert the cannula, length of catheter, indwelling time, presence of underlying atherosclerosis, and use of vasopressors. Arterial catheterization also may result in arterial injury and lead to local hematoma or pseudoaneurysm formation. The risk of pseudoaneurysm formation is increased with local infection. Infectious complications of arterial lines are rare but may be related to cannulation site, and in particular the femoral site, where infection may occur in up to 18% of cases. Therefore all arterial cannulation insertions should be conducted with full sterile technique.
Because the oscillometric method is not standardized and measuring algorithms are protected by each manufacturer, it is difficult to make accurate comparisons between both methods. Several studies have shown discrepant results between methods in critically ill patients. Oscillometry generally underestimates intra-arterial systolic blood pressure, as opposed to diastolic blood pressure, which is overestimated, particularly in hypertensive patients. MAP measurement is more reliable.
The invasive blood pressure monitor must be zeroed and leveled, in the phlebostatic axis (at the level of the right atrium), regardless of the site of the arterial cannula. Inadequate or incorrect zeroing results in incorrect diagnoses and inappropriate therapy. The bedside clinician should always check the “zero” before initiating antihypertensive or vasopressor therapy.
Pulse Pressure and Stroke Volume Variability
A major reason to use invasive rather than noninvasive blood pressure monitoring in the ICU is to use respiratory cycle–varying measurements of stroke volume variability (SVV) and pulse pressure variation (PPV) during mechanical ventilation to help predict fluid responsiveness. Indeed some studies suggest that SVV may be the most accurate predictor of fluid responsiveness, and therapy that is based on SVV appears to be associated with improved hemodynamic stability. Arterial contour analysis has been validated against indirect measures of cardiac output using thermodilution or dye dilution techniques. Positive intrathoracic pressure increases right ventricle (RV) afterload and reduces RV preload. Hence, on inspiration, RV stroke volume falls and results in a decrease in the subsequent left ventricle (LV) stroke volume, which manifests during the next expiration. In addition, LV stroke volume may increase slightly during inspiration as a consequence of increased LV filling from enhanced pulmonary venous return, increased LV compliance because of decreased RV dimensions, decreased LV afterload, and external pressure on the LV. Thus respiratory variations in arterial pressure reflect in large part the corresponding cyclic variations in LV stroke volume. This relationship has been demonstrated in directly measured (with transesophageal echocardiography [TEE]) SVV or through measurement of arterial PPV, a surrogate of SVV. PPV is a good indicator of fluid responsiveness as can be demonstrated when plotting stroke volume against end diastolic volume on a Frank-Starling curve. Variations are exaggerated when the LV is functioning on the steep portion of the curve, whereby small changes in preload induce large changes in stroke volume. Patients on the flat portion of the curve are insensitive to these cyclic respiratory-related changes in preload ( Fig. 19.1 ).

In the mechanically ventilated patient, the effect of respiration on blood pressure can be measured by adjusting the speed of the arterial pressure to match the end-tidal carbon dioxide (etCO 2 ) (or airway pressure) trace on the monitor. A 15% decrease in pulse pressure appears to be a sensitive indicator of fluid responsiveness. PPV or SVV has been shown to predict fluid responsiveness in mechanically ventilated patients, in cardiac surgery patients, in septic shock, with hemorrhage, and in elective brain surgery. However, these findings are not universal and other studies have failed to demonstrate a clear relationship between SVV correlates and fluid responsiveness.
Unlike bedside echocardiography, arterial pulse contour analysis does not require an experienced operator and its use is associated with less patient discomfort. However, the arterial pressure contour may not always predict stroke volume because the pulse contour analysis omits the contributions of changing vascular tone and vascular bed distribution. In addition, several limitations summarized by the acronym SOS need to be considered when using SVV: (1) S, small tidal volume, for example, lung protection strategies and spontaneous breathing activity; (2) O, open chest conditions; and (3) S, sustained cardiac arrhythmia because all may influence the accuracy of SVV evaluation of volume status or fluid responsiveness. Thus respiratory cycle-varying measurements related to cardiac function may be useful tools in the management of neurocritical care unit (NCCU) patients, particularly those that have large fluctuations in circulating volume (e.g. patients that have diabetes insipidus or those receiving aggressive osmotherapy with resultant diuresis).
Monitoring Flow and Volume
To maintain homeostasis, organs require blood flow sufficient to match metabolic demands. There are two components to flow: volume and pressure. There must be sufficient volume in the circulation to ensure that the entire organ tissue receives distribution, and this must be driven by adequate pressure to ensure its timeliness. So in simple terms, flow is volume divided by time. In general, perfusion pressure is proportional to flow, and pressure divided by flow equals resistance. However, if only pressure (in this case blood pressure) is measured, misleading conclusions about flow may arise: high resistance results in normal pressure but low flow ( Fig. 19.2 ). Hence, blood pressure alone should be used with caution as a surrogate for tissue flow. For example, the kidney, an autoregulated organ, is highly sensitive to hypoperfusion, which is manifested as oliguria. Hence, the combination of a “normal” blood pressure and a urinary output greater than 0.5 mL/kg/hr suggests adequate blood flow. However, oliguria may be associated with the stress response, and polyuria can result from diuretic administration, particularly mannitol.

Evidence of end organ perfusion is helpful if present but means to measure it are relatively limited in routine care. What are the alternatives? Tissue blood flow can be measured using either specific flow monitors or laboratory analysis of end products of metabolism. Flow monitors measure stroke volume and cardiac output or analogous markers of cardiac function. Laboratory markers include by-products of metabolism, such as lactate, or oxygen consumption using, for example, mixed venous oxygen saturation (SVO 2 ). Other monitor types that measure tissue perfusion (such as tissue oxygen electrodes) or metabolic activity (such as carbon dioxide: capnometry) also may give information about flow.
Principles of Cardiac Output Monitoring
Because blood pressure monitoring allows for limited inference about blood flow, there is a widespread interest in measuring stroke volume and cardiac output (CO). This, at least theoretically, provides information about circulating volume, cardiac preload, afterload, and contractility and its response to volume loading, changes in pressure, and diuresis. This may be particularly useful in the brain-injured patient in whom cardiovascular dysfunction may accompany neurologic injury. Furthermore, the cardiovascular system matches blood flow to the body’s demands that may vary among patients and within the same patient over time. Therefore there is no single “normal” value of CO, and decisions about the adequacy of CO need to be considered with other variables (e.g., tissue perfusion and metabolic demand) in mind.
There are two types of cardiac output monitors: those that use thermodilution or the Fick principle to measure right ventricular stroke volume and those that analyze arterial waveforms to calculate CO. The thermodilution method uses a change in pulmonary (or femoral) arterial pressure to calculate CO, associated with injection of below body temperature fluid or the use of a heating coil. A thermistor in the artery generates a temperature change curve, the area under which is proportional to the CO, which can be calculated. This represents the gold standard method to measure CO; it is simple and reproducible. Thermodilution CO is the method used to measure cardiac output in pulmonary artery (flotation) catheters (PACs, Swan-Ganz catheters) and the PAC-based continuous thermodilution methods (Vigilance, Edwards Life Sciences [Irvine, CA]; Opti-Q, ICU Medical [San Clemente, CA]; and TruCCOMS, AorTech [Rogers, MN]). Less invasive devices also using thermodilution (e.g., PiCCO [Pulsion Medical Systems, Irving, TX] and VolumeView [Edwards Life Sciences, Irvine, CA]), ultrasound (e.g., COstatus [Transonic Systems Inc., Ithaca, NY] or lithium chloride as an indicator (e.g., LiDCO [LiDCO Ltd., Cambridge, UK]). These devices use central venous (for calibration) and peripheral arterial cannulas (sensor) instead of needing pulmonary artery cannulation. The PiCCO and VolumeView systems require a femoral artery catheter. Application of all dilution methods assumes three major conditions: (1) complete mixing of blood and indicator, (2) no loss of indicator between place of injection and place of detection, and (3) constant blood flow. The errors made are primarily related to the violation of these conditions. Of the mentioned methods the transpulmonary indicator dilution methods are, as the so-called continuous cardiac output thermodilution methods, reasonably accepted in clinical practice and have been used in neurocritical care. For example, Mutoh et al. compared PAC CO measurements to transpulmonary thermodilution using PiCCO-derived CO in patients with SAH. Pooled analysis of data showed close agreement between PAC and PiCCO-derived values of cardiac index.
According to the Fick equation, CO (in liters per minute) equals oxygen consumption (milliliters of O 2 per minute) divided by the arteriovenous O 2 difference (milliliters of O 2 per liter of blood). The NiCO (noninvasive cardiac output, Novametrix) device uses the Fick equation by calculating CO through changes in capnometry associated with re-breathing CO 2 through a dead space circuit within the breathing system.
Pulse contour analysis is based on the concept that the contour of the arterial waveform is proportional to the stroke volume, which can be estimated by the integral of the change in pressure from end systole to end diastole over time. Most arterial contour methods relate an arterial pressure or pressure difference to a flow or volume change (PiCCO [Pulsion], PulseCO [LiDCO] and Modelflow [TNO/BMI]). Because the estimated stroke volume is determined by the elastic and resistance patterns of the aorta, the devices are usually calibrated using transpulmonary thermodilution, typically with a femoral arterial catheter and a central venous line. Output of these pulse contour systems is calculated beat-to-beat, but presentation of the data is typically with a 30-second window.
The most sophisticated arterial waveform analysis tool is the esophageal Doppler monitor (EDM), which is placed transorally in the esophagus. The Doppler ultrasound measures the extent of distension of the thoracic aorta during systole, from which stroke volume and CO are derived.
Central Venous Pressure: What It Does and Does Not Do
Central venous catheters (CVCs) are 8- to 20-cm flexible catheters that are placed in a central vein (subclavian, internal jugular, or femoral), the tips of which lie in the superior vena cava (SVC), inferior vena cava (IVC), or right atrium. They are inserted for a variety of reasons: the administration of vasopressors and hypertonic dextrose solutions (total parenteral nutrition), long-term venous access, and measurement of central venous pressure (CVP) among them. Peripherally inserted central catheters (PICCs) are longer lines typically inserted in antecubital veins with their tips also in the SVC. PICC lines have replaced CVCs for drug and nutrition delivery, but they are considered unreliable to monitor caval pressures. Nonetheless, even CVP measurements obtained from CVCs remain a controversial method to estimate effective circulating volume. There is no “normal” CVP, and small changes in its magnitude may result from large changes in circulating volume. However, CVP measurement may still be valuable because it may indicate a change in patient physiology, and changes in the waveforms may reflect intrinsic cardiac abnormalities ( Fig. 19.3 ). CVP is useful as a trend and should be combined with some form of flow monitoring such as SVO 2 , stroke volume, urine output, or mentation. In this paradigm, the CVP is used to construct Starling curves.

Ventricular performance from volume loading usually reaches a plateau around a CVP of 10 mm Hg. When CVP is greater than 10 mm Hg, hypervolemia may be the cause, but other causes such as acute pulmonary hypertension, arrhythmia, RV dysfunction, cardiac tamponade, increased intrathoracic or intra-abdominal pressure (auto–positive end-expiratory pressure [PEEP], pneumothorax, abdominal compartment syndrome) should be considered and evaluated.
When CVP is elevated, interpretation of CVP waveforms can be useful. It is important to use electrocardiography to identify or time CVP waveforms. For example, the Y descent represents the decrease in atrial pressure at the beginning of diastole. It follows the V wave. The right atrial Y descent usually is increased in restrictive physiology, pericardial constriction, volume-overloaded right heart or right heart ischemia, or infarction. In cardiac tamponade it is small. A large V wave is seen in tricuspid regurgitation; this may be associated with RV failure secondary to volume overload or intense hypoxic pulmonary vasoconstriction. In atrial fibrillation the A wave disappears. In atrioventricular dissociation or junctional rhythm a tall cannon A wave is produced. In patients with diastolic dysfunction these arrhythmias can cause sudden hypotension, and analysis of CVP waveform can be diagnostic.
Devices Used to Monitor Cardiac Output: Strengths and Weaknesses
Pulse Contour Methods
Systolic ejection results in the propulsion of a stoke volume into the arterial tree and distention of the aorta and distal arteries. A characteristic waveform is produced that reflects the stroke volume and elastic properties of the arterial wall. The shape of the pulse waveform and the area under the curve can be mathematically related to the cardiac output. However, arterial compliance is neither consistent nor constant and there is great inter- and intrapatient variability. In addition, aortic valve regurgitation may decrease the accuracy of arterial pressure waveform analysis. Because compliance is the mathematical relationship between pressure and volume, external calibration of the pressure signal with an alternative cardiac output technique is required. Pulse contour devices—Pulse CO LiDCO (LiDCO+) (LiDCO Ltd.), PiCCO (Pulsion Medical Systems, Munich, Germany), and VolumeView (Edwards Life Sciences)—combine peripheral arterial pulse contour analysis to calculate stroke volume and use dilution or thermodilution cardiac output measurement to calibrate the system. Devices that analyze pulse waveforms also analyze and display pulse pressure variability, which may be used to assess dynamic preload and fluid responsiveness (in mechanically ventilated patients).
PiCCO and VolumeView calculate cardiac output continuously from pulse contour analysis of the aortic waveform via femoral arterial cannula using transpulmonary thermodilution. Both require a CVC (internal jugular or subclavian vein) to calibrate cardiac output after ice cold fluid injections centrally being sensed by the thermistor in the femoral artery. The major advantage of this system over a PAC is that there is no requirement to catheterize the right heart. The PiCCO device measures the area under the aortic waveform; the systolic area is identified as that part of the waveform proximal to the dicrotic notch, and this is proportional to the stroke volume. Although beat-to-beat volumes are measured, these are averaged over 30 seconds to avoid inaccuracy associated with anomalous waveforms, interference, and extrasystoles. The continued accuracy of these monitors depends on the frequency of calibration using thermodilution. The manufacturer recommends recalibrations every 8 hours, and more frequently in unstable patients.
In PiCCO and VolumeView monitors the temperature differential detected using the arterial thermistor is composed of a series of exponential decay curves as the cold injectate passes through the various compartments of the circulatory system. Unlike the PAC, the receiver is located at a significant distance from the injector. Consequently, a series of exponential decay curves are constructed as the injectate passes along. Because the injectate is administered centrally and the temperature difference is measured in a proximal artery, the majority of the temperature change occurs in the intathoracic compartment. As a consequence, it is possible to obtain an intrathoracic blood volume index (ITBVI) and extravascular lung water index (EVLWI). This is a significant advantage to the PiCCO device because pulmonary edema commonly accompanies brain injury from acute lung injury, heart failure, or of neurogenic origin. Finally, in addition to SVV, the device also purports to measure global end-diastolic volume, and so permits the construction of Starling curves with volume titration. To date, this particular device appears to correlate very well with thermodilution techniques.
Lithium Dilution
Lithium chloride in low doses is a nontoxic substance that is not metabolized. When injected, its concentration is easily measured using a lithium selective electrode. Lithium dilution cardiac output is calculated from the area under the concentration–time curve when injected from a central line and measured peripherally. Injection through the antecubital vein appears to be as accurate as a central line. Pulse CO LiDCO (LiDCO+) combines pulse contour analysis with lithium dilution calibration.
The major disadvantage of LiDCO+ is the injection of lithium and the requirement for calibration of cardiac output at least every 8 hours. This is not required in PiCCO. In addition, in patients that are hyponatremic or have recently received neuromuscular blocking agents, the calibration data may be inaccurate. Data are unreliable with aortic valve disease or balloon counterpulsation therapy. The major advantage of LCO+ is that no specialized central or arterial line is required.
Noncalibrated Pulse Contour Waveform Analysis
FloTrac/Vigileo (F/V, Edwards Lifesciences) calculates continuous cardiac output (CCO), stroke volume (SV), SVV, and systemic vascular resistance (SVR) using pulse contour analysis obtained from a single sensor attached to an arterial line at any peripheral site. Unlike PiCCO or VolumeView, it does not require external calibration, a central line, or specialized catheter. Since its introduction in 2006, the device has seen several software upgrades, with progressive improvement in accuracy. There were concerns that early software versions underestimated cardiac output when there was increased arterial compliance and vasodilatory shock, but third-generation software was recently validated in septic patients. F/V combines rapid analysis in real time of the arterial pressure waveform with demographic data (such as sex, age, weight, and height) applied to an evolving algorithm to calculate cardiac output. Arterial pulsatility is directly proportional to stroke volume. The device appears capable of correcting for dynamic changes in vascular tone and compliance by analyzing skewness and kurtosis of the arterial waveform. These correction variables are updated every 60 seconds and the arterial waveform is analyzed and averaged over 20 seconds, thus eliminating artifacts, jitter, and premature contractions. Cardiac output is calculated using the arterial waveform and the heart rate yielding apparently reliable measures of SVV and hence fluid responsiveness.
Mayer et al. performed a meta-analysis of studies that have investigated F/V. Except for patients with irregular heart rhythms and aortic stenosis, cardiac output measurements by F/V are similar to those obtained using transthoracic echocardiography in critically ill patients. Earlier studies demonstrated poor correlation between F/V and thermodilution methods; with newer software the correlation has improved and with each software update accuracy continues to improve. It should be remembered that thermodilution methods, although considered the gold standard, are not ideal devices to compare with F/V; measurement intervals and averaging times are substantially longer with all thermodilution methods. Hence it is possible that F/V is more sensitive to dynamic changes in cardiovascular activity. Conversely, it is likely that the F/V is severely limited in patients with aortic valve disease, those with indwelling intra-aortic balloon pumps, those rewarming from hypothermia, and patients with intracardiac shunts. It is conceivable that this particular device will become the dominant product in the market in future because of its simplicity of placement, lack of observer error, continuity of data, and the lack of need for secondary vascular access. Currently, however, F/V is recommended in situations in which alternative monitors are unavailable. Another similar, noncalibrated monitor, the MostCare system (Vytech, Padova, Italy) uses the pressure recording analytical method (PRAM) to analyze the arterial wave contour peripherally. Similar to the other systems MostCare uses a proprietary algorithm to derive cardiac and flow variables from contour analysis.
Esophageal Doppler
Esophageal Doppler has been widely used in perioperative medicine (e.g., cardiac surgery), but has made minimal inroads into ICUs, particularly NCCUs. The thoracic aorta is located in proximity to the esophagus. The device uses Doppler ultrasound to measure aortic blood flow velocity, which allows derivation of SV and CO. Insertion of the probe is rapid, and clinically useful derived data are available within seconds. Substantial published data support the use of esophageal Doppler to guide goal-directed therapy. There are nonetheless several potential drawbacks: (1) patient positioning is awkward, (2) there is a steep learning curve and significant interobserver variability, and (3) the esophageal Doppler, although small in diameter and pliable, cannot be inserted into nonsedated and nonintubated patients or patients with known esophageal disease. This device is unlikely to find a place during neurosurgical procedures because the head may be inaccessible during surgery. To be used in NCCUs, placement problems and observer error need to be overcome.
Other Devices
NiCO (noninvasive CO [Novametrix; Wallingford, CT]) is a device that uses partial rebreathing of CO 2 to derive cardiac output in mechanically ventilated patients. The effects of unknown ventilation or perfusion inequality in certain patients may explain why the performance of this method shows a lack of agreement between thermodilution and CO 2 -rebreathing cardiac output. Furthermore, it may be unsuitable for neurologically injured patients because its use may cause fluctuation in CBF.
COstatus (Transonic Systems Inc., Ithaca, NY) calculates cardiac output by using ultrasound technology to measure changes in blood ultrasound velocity and blood flow following an injection of warm saline solution. Some studies have demonstrated that CO values measured with ultrasound indicator dilution techniques correlate well with PAC, and the COstatus system provides derived variables including total end diastolic volume index.
Pulse dye densiometry (Nihon Kohden, Japan) is a technique that uses transpulmonary dye dilution with transcutaneous signal detection to measure CO. The dye is injected intravenously, and although the system is relatively noninvasive, supporting data for this technology is limited. This approach cannot be currently recommended.
Electrical bioimpedance uses constant electrical current stimulation to identify thoracic impedance variations associated with blood flow. Bioreactance has evolved from bioimpedance and measures changes in frequency of electrical currents across the chest wall. These concepts are noninvasive and elegant in design, but to date there are few data to support the use of this approach in critical care. In addition, electrical interference may limit their potential use in the ICU.
Right Heart Catheterization and Pulmonary Artery Pressure Monitoring
The balloon tipped flow-directed PAC was introduced in the early 1970s and quickly became widely used in critical care, under the moniker of its inventors Swan and Ganz. The bedside ability to “float” a catheter into the heart originated on a serendipitous observation by cardiologist H. J. Swan when he observed a sailboat and imagined a parachute-like device catching blood flow and dragging a catheter with it. Swan subsequently worked with William Ganz to incorporate cardiac output computation capability using thermodilution into the catheter itself. Despite decades of controversy about whether information provided by a PAC can help influence outcomes in ICU patients, use of the PAC has declined significantly, particularly outside of North America. Nevertheless the PAC remains the gold standard hemodynamic monitor in clinical use to assess cardiac output, and despite its limitations most other devices are compared to it. In addition a PAC also can provide information about pulmonary artery pressures, right-sided and left-sided filling pressures, and SVO 2 . In general, its use should be reserved for more complex patients.
The PAC directly measures intrathoracic, intravascular, and intracardiac pressures and is able to display their pressure waveforms. It also provides measurement of CO and SVO 2 . Measurement of these hemodynamic indices is continuous and mostly accurate and clearly superior to the clinical assessment of these indices by physical examination in critically ill patients.
The PAC can be inserted through a wide bore introducer in any central vein, and “floated” through the right atrium and ventricle into one of the pulmonary arteries using a balloon attached to the distal part of the catheter. Usually the device is advanced until the balloon is “wedged” into a proximal arteriole, at which point flow ceases and the port distal to the balloon is able to measure the static pressure in the fluid column that equilibrates across the pulmonary capillaries and left atrium. Thus because there are no valves between the pulmonary capillaries and the left atrium, this pulmonary capillary wedge pressure (PCWP) is a reflection of left atrial pressure ( Fig. 19.4 ).
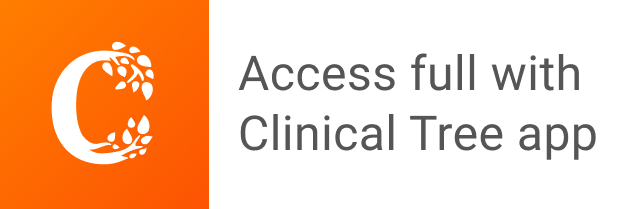