Adenosine Metabolism
Abstract
Adenosine is a neuromodulator that plays a role in energy metabolism, cellular communication, and DNA methylation. Purinergic signaling can be carried out by two different families of receptors: adenosine (P1) and nucleotide (P2X and P2Y) receptors. Levels of available adenosine in the brain are regulated by various metabolic enzymes, including adenosine kinase (ADK), 5ʹ-nucleotidase (5ʹ-NT), and adenosine deaminase (ADA). Expression levels of these proteins, however, are altered in epileptic tissue. For example, ADK expression is upregulated, resulting in faster metabolism and clearance of adenosine. Adenosine can modulate neuronal activity and exhibits natural antiepileptic effects through its activation of A1 receptors. A reduction in A1 receptor expression, however, is commonly found in epileptic tissue. The combination of these changes diminish the natural anticonvulsant ability of adenosine. Changes in purinergic signaling and metabolism in epilepsy as well as the therapeutic potential of targeting and altering adenosine metabolism will be discussed.
Keywords
Adenosine kinase; 5ʹnucleotidase; P2X7 receptors; adenosine augmentation therapy (AAT); adenosine polymers; DNA methylation; P1 receptors; P2 receptors; ketogenic diet
Overview
The purine nucleoside adenosine plays a neuromodulatory role in the brain. It is directly involved in a number of functions including metabolism, cellular communication, and DNA methylation. Purinergic signaling by adenosine and its metabolites can occur through a variety of receptors and have profound intracellular effects. Therefore, it is crucial to maintain homeostatic adenosine levels in the brain. This is accomplished by regulatory metabolic enzymes, including adenosine kinase, 5’-nucleotidase (5’-NT), and adenosine deaminase (ADA). Expression levels of these proteins, however, are altered in epileptic tissue. For example, ADK expression is upregulated, resulting in faster metabolism and clearance of adenosine. Adenosine can modulate neuronal activity and exhibits natural antiepileptic effects through its activation of A1 receptors. A reduction in A1 receptor expression, however, is commonly found in epileptic tissue. The combination of these changes diminish the natural anticonvulsant ability of adenosine. In this chapter, we will review changes in purinergic signaling and metabolism in epilepsy. We will also discuss the therapeutic potential of targeting and altering adenosine metabolism, adenosine-regulated glutamate release, DNA hypermethylation, and the effects of a low glycemic diet.
Purinergic Signaling and Metabolism
Adenosine is a ubiquitous purine neuromodulator that is directly involved with energy metabolism and cellular communication. Purinergic signaling can be carried out by two different families of receptors: adenosine (P1) and nucleotide (P2X and P2Y) receptors (Table 3.1). P2X receptors (P2X1-P2X7) are ligand-gated ion channels whereas P1 and P2Y receptors are G-protein-coupled receptors (GPCRs, Table 10.1) [1–3]. Activation of P1 or P2Y receptors may inhibit cyclic adenosine monophosphate (cAMP, Gαi), stimulate cAMP (Gαs), or activate phospholipase C (Gαq) and subsequently lead to both the activation of protein kinase C (PKC) and the release of internal calcium stores. Glial cellular distribution of the purinergic receptors is detailed in Table 10.2 [4]. A comprehensive overview of the cellular distribution of purinergic receptors can be found in Burnstock and Knight [5].
Table 10.1
Purinergic G-Protein-Coupled Receptor Classification
Receptor Family | Receptor Subtype | Gα Subunit Subtype |
P1 | A1 | Gαi |
A2A | Gαs | |
A2B | Gαs | |
A3 | Gαi and Gαq | |
P2Y | Y1 | Gαq |
Y2 | Gαq | |
Y4 | Gαq and Gαi | |
Y6 | Gαq | |
Y11 | Gαq and Gαs | |
Y12 | Gαi | |
Y13 | Gαi | |
Y14 | Gαi |
Table 10.2
Purinergic Receptors in Glial Cells
Receptor | Astrocyte | Müller (eye) | Enteric Glia | Schwann Cells | Oligodendrocytes | ||||
Myelin | Nonmyelin | Terminal | Progenitor | Myelin | Microglia | ||||
ADENOSINE | |||||||||
A1 | R, P, F | F | R | P, F | F | ||||
A2A | F | R, P, F | R | F | |||||
A2B | F | R | R | F | |||||
A3 | F | R | F | ||||||
ATP (IONOTROPIC) | |||||||||
P2X1 | R, P, F | R | |||||||
P2X2 | R, P, F | ||||||||
P2X3 | R, P, F | ||||||||
P2X4 | R, P, F | R | R, P, F | ||||||
P2X5 | R, F | R | P | ||||||
P2X6 | R, P | P | |||||||
P2X7 | R, P | R, P, F | P | F | P, F | F | P, F | ||
ATP (METABOTROPIC) | |||||||||
P2Y1 | R, P, F | F | F | R, F | |||||
P2Y2 | R, F | F | F | F | F | F | F | ||
P2Y4 | R, F | F | F | R, F | |||||
P2Y6 | R, F | F | R, F | ||||||
P2Y11 | F | ||||||||
P2Y12 | F | R, F | |||||||
P2Y13 | F | R | |||||||
P2Y14 | F |
Reproduced with permission from Fields RD, Burnstock G. Purinergic signalling in neuron-glia interactions. Nat Rev Neurosci 2006;7(6):423–36.
Various enzymes are involved in the metabolism of adenosine (Fig. 10.1) [6]. Adenosine kinase (ADK) phosphorylates adenosine to form AMP. Initially, ADK is predominantly expressed in neurons but by postnatal day 14 in rats, ADK expression migrates to an almost exclusively astrocytic distribution [7]. The major adenosine producing enzyme in the extracellular space is 5ʹ-nucleotidase (5ʹ-NT); it conducts the reverse reaction by hydrolyzing AMP to adenosine. Equilibrative nucleoside transporters (ENTs) can move nucleoside substrates, including adenosine, into the cell. Adenosine may be deaminated by the enzyme adenosine deaminase (ADA). More specifically, ADA catalyzes the deamination of both adenosine and 2ʹ-deoxyadenosine to inosine and 2ʹ-deoxyinosine, respectively, while producing ammonia as a byproduct [8]. Altogether, these enzymes can regulate the total amount of available intracellular and extracellular adenosine in the brain.

Under physiological conditions, a major source of synaptic adenosine is vesicular release of ATP (orange circle) from astrocytes followed by its extracellular degradation to adenosine (ADO) by a cascade of ectonucleotidase (EN). Under conditions of high-frequency stimulation neurons contribute to synaptic ATP release. Extra- and intracellular levels of adenosine are rapidly equilibrated, mainly by equilibrative nucleoside transporters (ENTs). Thus, synaptic concentrations of adenosine are largely dependent on its intracellular metabolism as the driving force for the influx of adenosine into the cell. Intracellular metabolism of adenosine depends on the activity of the astrocytic enzyme adenosine kinase (ADK), which, together with 5′-nucleotidase (5′-NT), forms a substrate cycle between AMP and adenosine. This substrate cycle is directly linked to the energy pool of the brain involving ADP and ATP. Source: Reproduced with permission from Boison D. The adenosine kinase hypothesis of epileptogenesis. Prog Neurobiol 2008;84(3):249–62.
Transmethylation is an enzymatic reaction characterized by the transfer of a methyl group. Adenosine is the end product of S-adenosylmethionine (SAM)-dependent transmethylation reactions. The final reaction in this process involves the hydrolysis of S-adenosylhomocysteine (SAH) to homocysteine and adenosine by the enzyme S-adenosylhomocysteinase. From there, homocysteine can be recycled back to methionine via a methyl transfer reaction and subsequently converted back to SAM. Interestingly, increased levels of ADK may lead to hypermethylated DNA by removing adenosine and driving the flux of methyl groups through the transmethylation pathway (Fig. 10.2, left panel) [9]. Both the reduction of ADK expression and the increase in adenosine levels shifts the equilibrium of S-adenosylhomocysteinase toward the formation of SAH, which blocks DNA methylation by DNA methyltransferase [9]. Therefore, adenosine is an epigenetic regulator of DNA methylation.

Increased adenosine kinase (ADK) expression drives increased DNA methylation as a prerequisite for progressive epileptogenesis. Conversely, adenosine therapy restores normal DNA methylation and thereby prevents epileptogenesis. SAM, S-adenosylmethionine; SAH, S-adenosylhomocysteine; DNMT, DNA methyltransferase; 5mC, 5-methyl-cytidine. Source: Reproduced with permission from Boison D. Adenosinergic signaling in epilepsy. Neuropharmacology 2016;104:131–9.
Adenosine Metabolism and P1 Receptors in Epilepsy
Adenosine triphosphate (ATP) and adenosine are released during seizures [10] and the levels of both can be regulated through a number of metabolizing enzymes. Epileptic tissue has increased levels of 5ʹ-NT, which leads to increases in adenosine [11]. Once in the extracellular space, adenosine acting through all four P1 adenosine receptors (A1, A2A, A2B, and A3) plays a role in the modulation of synaptic transmission by controlling neurotransmitter release and fine-tuning synaptic activity [12,13]. Adenosine can be neuroprotective through the activation of inhibitory A1 receptors, although the expression levels of A1 receptors may be altered in epileptic tissue [9,12,14]. Adenosine can be cleared from the extracellular space by equilbrative nucleotide transporters (ENTs) or metabolically by ADK, levels of which are upregulated in epileptic tissue (Fig. 10.3) [14]. Evidence for alterations in adenosine metabolism and P1 receptor expression in both human epileptic tissue and animal models of epilepsy are discussed.

Nonpathological state (left panel): Extracellular adenosine (ADO) arises from two sources including: (1) Ca2+-mediated release of ATP from astrocytes followed by catabolism to ADO through a series of ectoenzymes that include nucleoside triphosphate diphosphohydrolases, ectonucleotide pyrophosphatase/phosphodiesterases and 5′-nucleotidases; and (2) Direct ADO release into the extracellular space upon postsynaptic stimulation of neurons. Once in the extracellular space ADO inhibits neuron excitation through activation of A1 receptors (A1Rs) localized to the pre- and postsynaptic neuron membrane. On the presynaptic neuron, A1R activation inhibits Ca2+-dependent vesicular release of excitatory neurotransmitters by inhibiting P/Q- and N-type voltage gated Ca2+ channels; while on the postsynaptic neuron A1R hyperpolarizes the cell by activating G-protein-coupled inwardly rectifying K+ channels (GIRKS). ADO is cleared from the extracellular space by passive propagation through concentrative and equilibrative transporters (ENT1/ENT2) on the astrocyte membrane. Once in the astrocyte cytoplasm ADO is metabolized into AMP by ADK, which sets the ambient ADO tone. Pathological state (right panel): Sustained neuronal excitation, as observed in epilepsy, induces a shift in adenosine receptor expression levels with A1R being superseded by A2A receptors (A2ARs). As a consequence, there is a loss of A1R activity, which translates to increased network excitability due to increased Ca2+-dependent vesicular release of excitatory neurotransmitters and attenuated GIRK-mediated hyperpolarization. Furthermore, A2AR activation causes an increase in astrogliosis that is accompanied by increased ADK expression and activity. Pathological levels of ADK will drive ADO influx and metabolism; thereby, decreasing the extracellular ADO tone. Source: Reproduced with permission from Aronica E, Sandau US, Iyer A, Boison D. Glial adenosine kinase—a neuropathological marker of the epileptic brain. Neurochem Int 2013;63(7):688–95.
Human Tissue Studies
Adenosine levels are elevated during a seizure and remain elevated during the postictal period [15]. ADK expression was increased in peritumoral tissue resected from patients with glial or glioneuronal tumors accompanied by epilepsy compared to tissue from seizure-free tumor patients [16]. Increased levels of ADK [17,18] and 5ʹ-NT [19] as well as decreased expression of the adenosine A1 receptor [20] were reported in tissue from patients with temporal lobe epilepsy (TLE). Proinflammatory molecules such as lipopolysaccharide (LPS) and interleukin-1β (IL-1β) increased ADK expression in cultured astrocytes from patients with TLE [17]. Membranes prepared from tissue resected from the temporal neocortex of patients with TLE or with epileptic cortical dysplasia were injected into Xenopus laevis oocytes [21,22]. In these oocytes and in neocortical slices from either human TLE tissue or pilocarpine-treated rats, GABA elicited inward currents [21]. Treatment with either ADA or nonselective adenosine receptor inhibitors reduced the GABA run-down current, a marker for GABAA-receptor instability [21]. The use of transgenic mice and selective antagonists revealed that A2A, A2B, and A3 receptors, but not A1 receptors, were involved in maintaining GABA current stability and thus fine-tuning neuronal excitability [21,22]. Altered GABA run-down currents were also observed in human neocortical slices of periglioma epileptic tissue [22].
Acute encephalopathy with biphasic seizures and late reduced diffusion (AESD) is a childhood encephalopathy characterized by a biphasic clinical course of severe febrile seizures and restricted diffusion in the subcortical white matter. In an analysis of single nucleotide polymorphisms (SNPs) in patients with AESD, genetic variation in the A2A receptor gene was a risk factor for AESD [23]. Similarly, certain SNPs in the A1 receptor [24], ADK [25], and 5ʹ-NT [25] genes were associated with developing seizures after traumatic brain injury (TBI).
Animal Models
The phenotype of astrogliosis (proliferation of and morphological alterations in “reactive” astrocytes), a common pathological feature of epilepsy, may be a result of adenosine largely acting through A2A receptors [6]. Both A2A receptors and ADK are upregulated in reactive astrocytes [9]. Selective A2A receptor antagonists prevented astrogliosis in rat primary astrocytes [26,27]. The use of in vitro systems determined that A2A receptor-dependent astrogliosis may occur through the basic fibroblast growth factor (bFGF) [26] or through the Akt/NF-κB [27] pathways.
It is well known that adenosine has powerful anticonvulsant effects [28–33]. Rat horizontal entorhinal cortex brain slices at least 2 months after electrical stimulation-induced status epilepticus (SE) exhibited increased sensitivity to adenosine compared to control slices [31]. Pharmacological evidence suggested that adenosine acts through the A1 receptor, but not the A2A receptor, to inhibit membrane excitability [7,31,33–36]. In fact, antagonist of the A1 receptor can accelerate seizure progression [7]. In the low magnesium in vitro entorhinal cortex slice preparation model of epilepsy, an adenosine A1 receptor antagonist accelerated the progression of seizure-like events (SLEs) into late recurrent discharges (LRDs, epileptiform activity that resembles SE) (Fig. 10.4) [28]; this could be reversed with an A1 receptor agonist (Fig. 10.5) [28]. Similarly, an inhibitor of 5ʹ-NT, which effectively depleted extracellular adenosine, increased the frequency of SLEs whereas an inhibitor of ADA, which raised extracellular adenosine, reversed these events [28]. A separate study, however, found that both ADA and S-adenosylhomocysteinase had no effect on neuronal activity in rat hippocampal slices [35]. ADK inhibitors also raised extracellular adenosine levels and reduced hyperpolarization and seizure-like activity in hippocampal slices from rats (Fig. 10.6) [7,35].

In (A), a slice that exhibited a stable, low frequency of SLEs during the previous 2 hours was exposed to a low concentration of DPCPX, shown by the arrow. Over the course of the 5–10 minutes wash in, a conversion of the epileptiform activity into LRD-like behavior occurred. The transition was associated with a gradual increase in frequency that reaches a maximum. A recovery of the DPCPX-induced switch was never observed, even following wash back to low Mg2+ alone for several hours. (B) The concentration dependence of the effects of DPCPX on the frequency of the events; the values are shown as a percentage change compared with control. Error bars represent SEMs. *represents a significance value of P <0.05, **represents a significance value of P <0.01 and ***represents a significance value of P <0.001 all compared with predrug values. All time scale bars in (A) represent 2 minutes; note the faster and slower chart speeds used. Vertical scale bar represents 0.5 mV. Source: Reproduced with permission from Avsar E, Empson RM. Adenosine acting via A1 receptors, controls the transition to status epilepticus-like behaviour in an in vitro model of epilepsy. Neuropharmacology 2004;47(3):427–37.

This slice (shown in (A)) had spontaneously switched its electrographic behavior to produce LRDs. Application of 0.1 μM CPA, the A1 receptor agonist, shown by the arrow, effectively converted the high-frequency activity back to the SLEs, note also the recovery in the amplitude of the events following CPA. This slice, despite washing back to low Mg2+ alone, showed no reversal of the SLEs back into LRDs even 60 minutes after wash back, suggesting a permanent recovery by CPA. The concentration dependence of the ability of CPA to reverse the activity is shown in (B), where even 0.01 μM CPA was effective. Error bars represent SEMs. **represents a significance value of P <0.01 and ***represents a significance value of P <0.001 all compared with predrug values. All time scale bars in (A) represent 2 minutes; note the faster and slower chart speeds used. Vertical scale bar represents 0.5 mV. Source: Reproduced with permission from Avsar E, Empson RM. Adenosine acting via A1 receptors, controls the transition to status epilepticus-like behaviour in an in vitro model of epilepsy. Neuropharmacology 2004;47(3):427–37.

(A) Epileptiform activity induced by high-frequency stimulation (HFS, small black bar) in nominally Mg2+-free artificial cerebrospinal fluid (aCSF) was greatly attenuated by the adenosine kinase (ADK) inhibitor iodotuberocidin (IODO, 5 mM; right panel) compared to control conditions in the absence of IODO (control; left panel). (B) The reversible depression of synaptic transmission induced by seizures under control conditions (filled squares) was also greatly attenuated in the presence of IODO (gray squares). Average duration of seizures (~18 seconds) in control conditions is indicated by gray bar, but too short to depict (~4 seconds) in the presence of IODO. (C) The IODO-induced depression of seizure activity (middle panel; IODO) is reversed by the A1 receptor antagonist CPT (1 mM; IODO/CPT; right panel). Note: Seizure in IODO/CPT is a spontaneous seizure, seen in 6/9 slices exposed to this combination of drugs. (D) Pooled data showing the inhibition of HFS-evoked seizure duration by IODO (n = 9) and its reversal by CPT in the 3 slices not showing spontaneous seizure activity. Source: Reproduced with permission from Etherington LA, Patterson GE, Meechan L, Boison D, Irving AJ, Dale N, et al. Astrocytic adenosine kinase regulates basal synaptic adenosine levels and seizure activity but not activity-dependent adenosine release in the hippocampus. Neuropharmacology 2009;56(2):429–37.
Pretreatment with the adenosine A3 receptor agonist IB-MECA increased seizure latency and decreased neurological impairment in N-methyl-D-aspartate (NMDA) and pentylenetetrazol (PTZ), but not electrically-induced seizures [37]. The mortality rate in all three models, however, was improved with IB-MECA treatment [37]. In immature rat hippocampal slices perfused with the GABAA-receptor antagonist bicuculline, however, the A3 receptor agonist 2-Cl-IB-MECA increased excitatory effects, including the frequency of spontaneous discharges [38]. These effects could be blocked by A3 receptor antagonists, but not by A1 or A2A antagonists [38]. The contrasting results could be explained by the excitatory actions of GABA in the immature brain.
Adenosine can decrease the firing rate of neurons [39]. Dialysate hippocampal purine levels were measured before and after electrically kindled seizures in rats in vivo [40]. While only a small increase in adenosine was observed, a two- to threefold increase in its metabolites (inosine, hypoxanthine, and xanthine) was found [40]. Decreased A1 receptor density was reported in the amygdala kindling model in rats [36] but no alterations in A1 receptor expression were reported in the rat perforant path stimulation model [41]. Application of the selective A1 receptor agonist CPA reduced the severity of seizures whereas the selective antagonist DPCX had no effect on severity or progression to SE [41].
A persistent upregulation in ADK protein expression in reactive astrocytes was observed for several months in the electrical stimulation [17] and kainic acid [42–44] models of TLE. An initial loss of ADK expression was reported after intrahippocampal kainic acid injections, but this gradually increased over time until it was both overexpressed and exhibited increased enzymatic activity compared to control mice [42]. Furthermore, seizures and astrogliosis remained focal and restricted to ADK overexpressing areas in the intraamygdala kainic acid model [43,44].
Increased levels of astrocytic A2A receptors [45] and 5ʹ-NT [46] were reported within 1 week of kainic acid injections. This increase was not observed, however, in amygdala kindled rats [46]. In both the hippocampus and cerebral cortex, increased levels of ATP, adenosine diphosphate (ADP), and adenosine monophosphate (AMP) were found anywhere from 2 to 50 days after either intraperitoneal kainic acid or pilocarpine injections [47]. Treatment with an A1 receptor antagonist reduced the latency to SE whereas an A1 agonist provided both anticonvulsant and neuroprotective effects in the rat pilocarpine model of epilepsy [48]. Activation of the adenosine A1 receptor also suppressed seizures in the intrahippocampal kainic acid model of epilepsy [49].
A single injection of the convulsant PTZ significantly increased adenosine uptake in the hippocampus, cerebellum, and cortex but decreased uptake in the striatum of mice [50]. Rats with increased ATP hydrolysis in synaptosomes from the hippocampus and cerebral cortex were more resistant to PTZ-induced seizures [51]. PTZ kindling led to decreased expression of the A1 receptor binding sites [52]. A decrease in the expression of A1 receptors would severely limit the anticonvulsant effects of adenosine.
Wistar Albino Glaxo/Rijswijk (WAG/Rij) rats develop spontaneous nonconvulsive seizures after 2 months of age and are therefore a genetic model of human absence epilepsy. Compared to age-matched Copenhagen Irish (ACI) control rats, cerebral A2A receptor expression was lower in the 1.5-month-old (preepileptic) WAG/Rij rats but was elevated in the epileptic 6-month-old WAG/Rij rats [53]. Exposure of a specific A2A receptor agonist to cortical and thalamic slices from the epileptic rats led to modulation of cAMP formation and stimulation of the MAPK pathway, an effect that was absent in the preepileptic WAG/Rij rats [53].
It is clear that reactive astrocytes have different properties than healthy ones, including upregulated ADK and A2A receptor expression. One explanation for these changes may be that these systems undergo “interdependent maladaptive changes” during the development of epilepsy [9]. If the adenosine A2A receptor becomes upregulated (and responds more efficiently to extracellular adenosine), then the upregulation of ADK may be triggered to limit adenosine availability. The now limited source of adenosine may then trigger increased A2A receptor expression, creating a self-sustaining cycle. This, however, does not explain the overall reduction in A1 receptor expression observed in epileptic tissue.
Transgenic Mice Studies
Purine inhibition of synaptic transmission is almost exclusively mediated by A1 receptor [54]. Intrahippocampal kainic acid injections led to increased neuronal cell loss, seizure severity, and seizure-induced fatality in A1 receptor knockout mice compared to wild-type mice [55]. After controlled cortical impact (CCI), seizures were observed in 83% of male and 100% of female A1 receptor knockout mice, 0% of male and 14% of female heterozygotes, and in 33% of male and 25% of female wild-type mice [56]. Seizure scores were higher in the A1 receptor knockout mice after CCI and lethal SE was only observed in knockout mice [56]. Adenosine A2A receptor knockout mice, on the other hand, were only partially resistant to PTZ- and pilocarpine-induced seizures but not electroshock-induced seizures [57].
Although ATP can be broken down by multiple ectoenzymes to degradation products (ADP, AMP, adenosine, and inosine), the primary source of adenosine during seizures may be from the breakdown of AMP by 5ʹ-NT [58]. Adenosine formation was almost completely absent in slices from 5ʹ-NT knockout mice. Furthermore, A1 receptor activation suppressed the spread of penicillin-induced seizures nearly twice as fast as in A1 receptor knockout mice or wild-type mice treated with A1 receptor antagonists [58].
Transgenic mice overexpressing ADK were more susceptible to brain injury. ADK was upregulated after seizures in the intraamygdala kainic acid model of epilepsy. Spontaneous seizures could be mimicked by simply overexpressing ADK or knocking out A1 receptors in mice [44,59]. Mice that overexpressed ADK died within 3 days of kainic acid-induced SE whereas mice with reduced expression of ADK in the forebrain were resistant to epileptogenesis (prevented astrogliosis and spontaneous seizures) [44,59]. Transgenic mice with overexpressed ADK in hippocampal neurons showed injury within 15 minutes of transient middle cerebral artery occlusion (MCAO); wild-type mice were spared from injury even after 1 hour of MCAO [60]. Wild-type mice had reduced hippocampal ADK expression after MCAO and reperfusion [60].
Adeno-associated virus 8 (AAV8) gene therapy vectors were used to selectively modulate ADK expression. ADK cDNA was put under the control of the astrocyte-specific promotor gfaABC1D [61] and viral vectors were injected into the CA3 area of wild-type or spontaneously epileptic ADK transgenic mice to selectively overexpress ADK in astrocytes. This led to focal overexpression of ADK and spontaneous seizures [62,63]. ADK downregulation with AAV8-mediated RNA interference (RNAi) almost completely abolished the spontaneous seizures in ADK transgenic mice [63].
Therapeutic Modulation of Adenosine
The regulation of adenosine metabolism offers ample therapeutic opportunities because adenosine, acting through the A1 receptor, is a natural anticonvulsant [12,64–66]. A number of different enzymes play a role in controlling adenosine levels (Fig. 10.1) and all have the potential to serve as therapeutic targets. ADK, however, is a major negative regulator of adenosine levels and is found predominantly on astrocytes. Other membrane proteins, including the A2A receptor and ENT, have been linked to controlling extracellular glutamate levels through inhibition of glutamate transporter-1 (GLT1). Glutamate has powerful effects on neuronal excitability and, therefore, needs to be tightly regulated. Furthermore, DNA hypermethylation is linked to epilepsy and adenosine is known to play a major role in epigenetics by altering DNA methylation states. Finally, adenosine levels can potentially be regulated by a change in diet. Each of these therapeutic options will be discussed below.
Adenosine Metabolism
The known effects of adenosine in regulation of neuronal excitability [29,30,32] have been tested for efficacy in various animal models of epilepsy. Adenosine analogs had anticonvulsant effects, including decreased seizure occurrence and shortened seizure duration, in the amygdala kindling [67], 4-aminopyridine (4-AP), PTZ, picrotoxin, kainic acid, and strychnine models of epilepsy [68]. Similarly, adenosine injected into the rat prepiriform cortex provided dose-dependent protection against bicuculline-induced seizures, an effect that was potentiated by treatment with an ADK inhibitor, adenosine transport blockers, or an ADA inhibitor [69].
Several enzymes involved in adenosine metabolism could be targeted to modify the levels of adenosine during epilepsy. Adenosine transporter inhibitors, such as the potent inhibitor of equilibrative transporter 1 (ENT1) cannabidiol, may increase extracellular adenosine and suppress seizures [70,71]. ADK is upregulated in reactive astrocytes in epilepsy and ADK inhibition increases adenosine levels [12]. Homozygous ADK knockout mutant mice, however, displayed microvesicular hepatic steatosis 4 days after birth and died within 14 days with a fatty liver [72]. Adenosine receptor agonists, particularly A1 receptor agonists, have also demonstrated anticonvulsant effects, but failed to undergo successful clinical development due to the severity of side effects at effective doses [73–75]. Furthermore, global application of either A1 receptor agonists or ADK inhibitors induces side effects, including cardiovascular problems [12,66]. Therefore, focal adenosine augmentation therapies (AATs) are a promising approach for the suppression or even prevention of seizures [64–66,76].
ADK inhibitors increase adenosine concentrations in vivo and in vitro [73,77–79]. Fibroblasts engineered to release adenosine by inactivating both ADK and ADA in culture were then encapsulated into semipermeable polymers and grafted into brain ventricles [80]. This treatment paradigm offered nearly total protection from behavioral seizures and afterdischarges; control rats had full tonic-clonic convulsions. One major drawback, however, was that the encapsulated cells had a limited longevity and, therefore, seizure suppression was limited to 2 weeks [80].
A promising focal AAT was intraventricular grafting of adenosine-releasing cells encapsulated in a synthetic polymer. This therapy significantly reduced the number of severe seizures as well as the amplitude and duration of afterdischarges [81–83]. Shortly thereafter, embryonic stem cells (ESCs) were engineered to release adenosine by genetic disruption of ADK and were differentiated into neural precursor cells [84]. The ESCs were then injected into the rat hippocampus and prevented the development of seizures in the electrical kindling model of epilepsy [84].
Lentiviral RNAi was used to mediate the downregulation of ADK in human mesenchymal stem cells (hMSCs). These cells were then transplanted into the hippocampi of mice 1 week prior to intraamygdala kainic acid-induced SE [85]. Mice receiving ADK knockdown implants displayed a 35% reduction in seizure duration and a 65% reduction in neuronal cell loss compared to sham-treated and scrambled miRNA control mice (Fig. 10.7) [85]. Silk-based brain implants containing these same hMSCs also significantly reduced the number and duration of kainic acid-induced seizure (Fig. 10.8) [86].

Representative micrographs of the hippocampal formation from coronal brain sections taken from sham-treated control mice (SHAM), recipients of scrambled control miRNA expressing hMSCs (SC), or H239 human mesenchymal stem cell (hMSC) graft recipients 24 hours after intraamygdaloid injection of 0.3 μg KA. Sections were stained either with cresyl violet (A, B, D, E, G, H, J, L) or with TUNEL (C, F, I, L green). (A–C) Typical apoptotic cell death in the CA3 region of the hippocampus of sham controls ipsilateral (ipsi) to the KA-injected amygdala. (D–F) CA3-selective apoptotic cell death in scrambled control-graft recipients was comparable to the sham-treated control animals. (G–I) H239 hMSC graft recipients show markedly decreased ipsilateral cell loss, which becomes evident by a hardly discernible lesion within the stratum pyramidale and by a prominent reduction in the number of TUNEL positive cells (I). (J–L) Sham- and scrambled control like CA3 injury in H239 graft recipients in which KA was paired with selective A1 receptor antagonist DPCPX (1 mg/kg, intraperitoneal). Scale bars: A, B, D, E, G, H, J, K: 100 μm; C, F, I, L: 25 μm. Source: Reproduced with permission from Ren G, Li T, Lan JQ, Wilz A, Simon RP, Boison D. Lentiviral RNAi-induced downregulation of adenosine kinase in human mesenchymal stem cell grafts: a novel perspective for seizure control. Exp Neurol 2007;208(1):26–37.

(A) Recordings from a montage using a bipolar electrode inserted into injured CA3 (H1; H2) and a cortical ground/reference electrode (R). H1-R and H2-R traces show hippocampus/cortical recordings whereas trace H1-H2 shows intrahippocampal recordings (ie, between the two tips of the bipolar electrode). Sham-treated controls were compared with recipients of H239 hMSCs before (middle) or after (bottom) injection of the A1 receptor antagonist DPCPX (1 mg/kg, intraperitoneal). (B and C) Average number of seizures per hour and average seizure duration in respective treatment groups. Data are based on n=6 animals for each group and 16 hours of continuous EEG monitoring. Data are presented as ±SD and were analyzed using one-way ANOVA compared to sham. *P <0.001, #P >0.05 compared to sham control. Source: Reproduced with permission from Li T, Ren G, Kaplan DL, Boison D. Human mesenchymal stem cell grafts engineered to release adenosine reduce chronic seizures in a mouse model of CA3-selective epileptogenesis. Epilepsy Res 2009;84(2–3):238–41.
Syzbala et al. [87] designed and implanted adenosine-loaded polymers into the hippocampal fissure of rats ipsilateral to the site of hippocampal electrical kindling. These were filled with adenosine-containing microspheres, coated with multiple macroscale adenosine-loaded silk films, and covered with a silk-based capping layer. The polymers released 1 μg adenosine per day for 10 days. Animals implanted with the adenosine-releasing implant were completely protected from seizures over the 10 days of treatment while rats receiving a control implant developed severe seizures (Fig. 10.9) [87]. After the initial 10 days of treatment, the adenosine polymers still offered protection and a significant reduction in seizure development [87].

(A) Four days after infrahippocampal implantation of silk-based polymers with daily target release rates for adenosine of 0 ng (N = 5, red), or 1000 ng (N = 8, blue) kindling stimulations were delivered at a rate of 6 stimulations per day on days 4, 6, 8, and 11 following implantation. A total of 24 kindling stimulations were delivered. On day 12, the A1 receptor antagonist DPCPX (1 mg/kg, i.p.) was injected 30 minutes prior to stimulation. Each animal was tested again on day 13 (no DPCPX). Seizure stages were averaged across animals from each group for each individual stimulus. Note that recipients of a target dose of 1000 ng adenosine per display significant protection from kindling development, while DPCPX does not increase the seizure score. Errors are given as ±SD. Data were analyzed by two way ANOVA followed by a Bonferroni test; the significance of interaction between groups was determined as F = 6.704, P < 0.0001; significance levels of individual tests are indicated: *P < 0.05, **P < 0.01, ***P < 0.001. (B) 4 days after infrahippocampal implantation of silk-based polymers with daily target release rates for adenosine of 0 ng (N = 7, red), or 1000 ng (N = 5, blue) kindling stimulations were delivered at a rate of six stimulations per day on days 4, 5, 6, 7, and 8 following implantation. A total of 30 kindling stimulations were delivered. Please note the increased kindling frequency compared to (A). After the 30th kindling stimulation, kindling was discontinued for 9 days. Kindling stimulations were resumed at day 18. Seizure stages were averaged across animals from each group for each individual stimulus. Note that recipients of a target dose of 1000 ng adenosine per day resumed kindling at day 18 at a level at which kindling was discontinued at day 8. After 7 consecutive stage 5 seizures kindling was discontinued in control animals due to animal welfare considerations. Errors are given as ±SD. Data were analyzed by two way ANOVA followed by a Bonferroni test; the significance of interaction between groups was determined as F = 19.36, P < 0.0001; significance levels of individual tests are indicated: **P < 0.01, ***P < 0.001. Source: Reproduced with permission from Szybala C, Pritchard EM, Lusardi TA, Li T, Wilz A, Kaplan DL, et al. Antiepileptic effects of silk-polymer based adenosine release in kindled rats. Exp Neurol 2009;219(1):126–35.
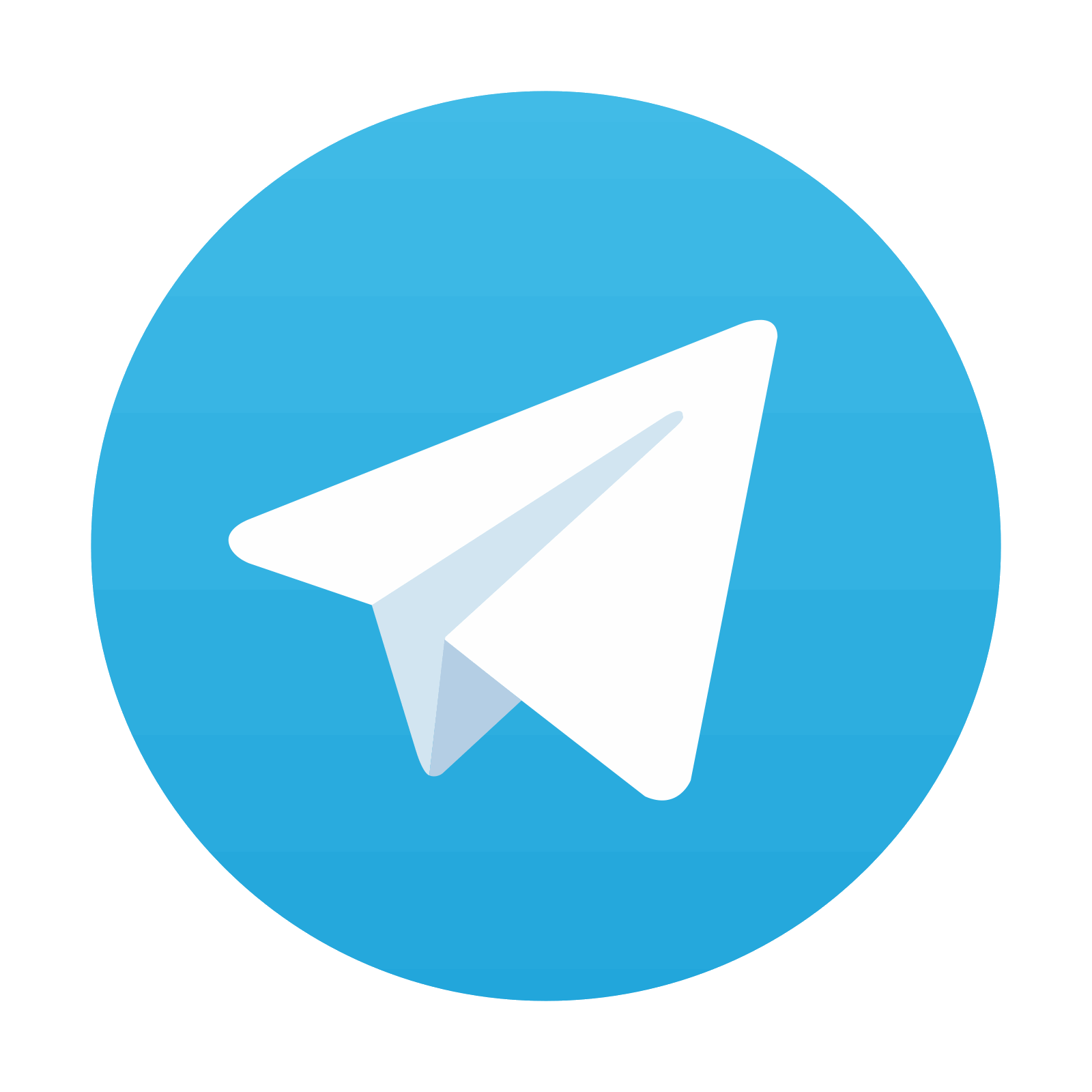
Stay updated, free articles. Join our Telegram channel
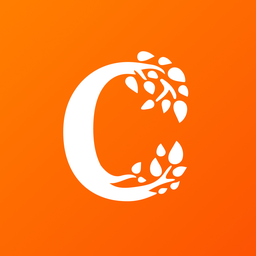
Full access? Get Clinical Tree
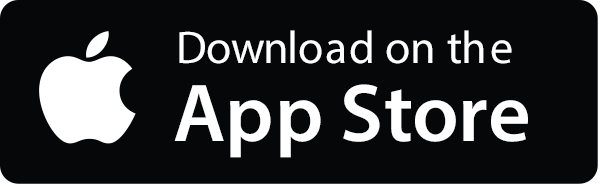
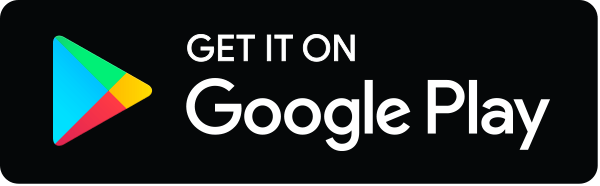
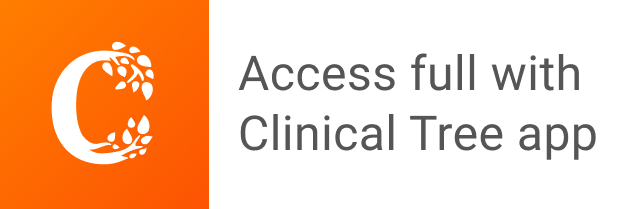