Astrocyte Calcium Signaling
Abstract
Several lines of evidence have pointed toward calcium-dependent exocytosis as a common mechanism of gliotransmitter release from astrocytes. Initial studies found increased intracellular calcium levels within astrocytes that were oscillatory and propagating. These calcium rises led to gliotransmitter release through a metabotropic glutamate receptor-dependent mechanism. Evidence for the presence of vesicular release proteins in astrocytes combined with pharmacological studies suggested an exocytosis mechanism of gliotransmitter release similar to that seen in neurons. The acceptance of this mechanism, however, became controversial after studies using transgenic mice demonstrated that increased astrocytic calcium had no effect on neighboring neurons. Astrocytic calcium signaling is altered in epilepsy. Increased intracellular calcium levels in astrocytes may lead to hyperexcitability and propagation of seizures. More in vivo studies are needed to fully understand the contribution of astrocyte calcium signaling to neuronal activity under physiological and epileptic conditions.
Keywords
Metabotropic glutamate receptors; slow inward currents; calcium-dependent exocytosis; epilepsy; seizure; calcium; astrocyte
Overview
Astrocytes may release gliotransmitters in a calcium-dependent manner. Specifically, the mechanism is thought to mimic the vesicular release of neurotransmitters seen in neurons. Evidence for this includes: 1) Astrocytes respond to glutamate with propagating calcium waves; 2) Metabotropic glutamate receptors (mGluRs) are present on astrocytes and likely play a role in calcium signaling; 3) Stimulation of astrocytic calcium elevations leads to gliotransmitter release; and 4) Vesicular machinery necessary for exocytosis is expressed in astrocytes. Despite this growing body of evidence, calcium-induced exocytosis in astrocytes remains highly controversial. While the role of astrocyte calcium signaling is not fully understood, current evidence suggests that it is altered in the epileptic brain. More specifically, dysregulation of calcium channels and increased astrocytic calcium signaling have been observed in epileptic tissue. In this chapter, we summarize the evidence and controversy surrounding calcium-dependent gliotransmission and the role of astrocyte calcium signaling in epilepsy.
Calcium-Induced Exocytosis
Several lines of evidence have pointed toward calcium-dependent exocytosis as a common mechanism for calcium signaling in astrocytes. Initial studies found oscillatory calcium waves within astrocytes that led to gliotransmitter release. This mechanism involves metabotropic glutamate receptors (mGluRs) and inositol triphosphate (IP3)-dependent release of internal calcium stores. Vesicular release proteins expressed by astrocytes suggested that gliotransmitters were released through exocytosis. Once released, gliotransmitters can then act on neighboring neurons and alter synaptic activity.
Elevated Intracellular Calcium in Astrocytes
Early evidence for astrocyte calcium signaling came from studies that demonstrated glutamate-triggered intracellular calcium wave oscillation and propagation in cultured astrocytes [1,2] and rat hippocampal slices [3–8]. Shortly after the initial discovery of glutamate-induced calcium waves in astrocytes [2], many other chemicals were found to produce the same effect including noradrenaline [9,10], histamine [11], acetylcholine [5,11,12], ATP [13–15], GABA [16], endocannabinoids [17], nitric oxide (NO) [18,19], and brain-derived neurotrophic factor (BDNF) [20]. Stimulation of mossy fibers originating in the dentate gyrus (DG) of rat organotypic hippocampal slice cultures led to delayed calcium waves in astrocytes within CA3 [21]. Long-lasting calcium oscillations were also seen after neuronal stimulation in both hippocampal and visual cortex transverse brain slices [22]. Propagating calcium elevations within astrocytes were induced by uncaging astrocytic IP3 [3] or mechanical stimulation of a single cell [1,23] and these waves were spatially restricted to functionally independent microdomains of astrocytes [24]. Nearby neurons also responded with increases in cytosolic calcium levels [22,23] which was blocked with the gap junction inhibitor octanol [23]. This suggests that the astrocyte-neuron communication occurred through intracellular connections rather than synaptic connections.
Parri et al. [25] discovered that astrocytes in rat ventrobasal (VB) thalamus slices displayed intrinsic intracellular calcium oscillations independent of action potential-evoked transmitter release and neuronal firing. Di Castro et al. [26] studied endogenous calcium activity in adult mouse hippocampal slice preparations and observed both focal (confined to a specific subregion) and expanded (spread to 9 or more contiguous subregions) events in astrocyte processes. In contrast to results presented by Parri et al. [25] in the VB thalamus, Di Castro et al. [26] found that while the focal events were dependent on synaptic neurotransmitter release, expanded events depended on nearby, individual action potentials. Calcium activity was abolished with an IP3 receptor blocker and overcome with subsequent bath application of thapsigargin (which empties intracellular calcium stores). Blocking astrocytic calcium activity decreased basal transmitter release at local synapses, suggesting that calcium elevations participate in local fine tuning of basal neurotransmission at excitatory synapses [26]. IP3 receptor subtype 2 (IP3R2) is the primary IP3 receptor expressed by astrocytes. IP3R2 knockout mice exhibited reduced calcium events [26], suggesting that activation of the IP3-sensitive calcium channel may be a major contributor to intracellular calcium release in astrocytes.
Additional calcium sources besides IP3 receptor-dependent release may contribute to cytosolic calcium levels within astrocytes. Diphenylboric acid 2-aminoethyl ester, a cell-permeable IP3 receptor antagonist, reduced mechanically induced calcium accumulation and glutamate release only by ~32% in astrocyte cultures [27]. Caffeine exposure increased internal calcium levels and this effect was reduced by ryanodine, a blocker of calcium release from caffeine/ryanodine-sensitive stores. Incubation with cadmium, a broad-spectrum antagonist of calcium entry into the cell, reduced calcium accumulation and glutamate release by ~55% [27]. These data suggest that both internal and external calcium stores are involved in gliotransmission.
Astrocytes display heterogeneity [28], including different pattern of calcium excitation depending on brain region [29–31]. Intracellular calcium elevations are not stereotyped signals; instead, they exhibit varied spatiotemporal patterns, likely underlying different types of functions and generating distinct output signals [32]. For example, glutamate released from stimulation of Schaffer collateral axons of hippocampal neurons evoked intracellular calcium increases in astrocytes that then elicited N-methyl-D-aspartate (NMDA) receptor currents in pyramidal neurons; glutamate released by alveus stimulation, however, did not [5]. This illustrates that astrocyte responses may discriminate the activity of different synapses or axon pathways.
Increases in astrocytic internal calcium levels leads to NMDA receptor-mediated slow inward currents (SICs) in neighboring neurons, likely due to the astrocytic release of glutamate [25,33–41]. This phenomenon has been observed in various brain regions, including the nucleus accumbens [35], dorsal horn [34], and the medial nucleus of the trapezoid body [39]. Pharmacological evidence suggests that these astrocyte-induced SICs are mediated by NR2B-containing NMDA receptors [35–37]. Furthermore, activation of protease-activated receptor 1 (PAR-1), but not P2Y1 receptor (P2YR1), led to SICs in pyramidal neurons under conditions that isolated the NMDA receptor response [41].
While the majority of studies on intracellular calcium changes in astrocytes have been conducted in vitro or in situ, only a few studies have explored this topic in vivo. Spontaneous intracellular calcium fluctuations were observed in the rodent cortex [42–46], hippocampus [40], and locus coeruleus [47]. Astrocytic calcium kinetics differs from those seen in neurons. Astrocytic calcium signals were oscillatory, often not synchronized, and exhibited a slow onset and subsequent plateau in elevation. Neurons, on the other hand, exhibited fast, transient calcium signaling with a single-exponential decay [44].
Metabotropic Glutamate Receptors
Gq-linked protein coupled receptor (GPCR) activation of phospholipase C leads to the release of IP3, activation of the IP3 receptor, and subsequent release of calcium from internal stores. Calcium imaging techniques were used to demonstrate that astrocytes in vitro and in situ respond to neuronal activity with GPCR-mediated intracellular calcium increases [5,6,12,16,22,26,32,33,48,49]. Specifically, neuronal stimulation led to activation of astrocytic mGluRs [6,17,22,40,49–51] and involved mGluR subtype 5 (mGluR5) [4]. It is likely that the astrocytic mGluRs are activated by glutamate released from neurons [6]. Stimulation of the somatosensory cortex led to increased astrocytic calcium, a response that was mediated by synaptic glutamate release and activation of mGluRs [45,46]. In response, astrocytes are capable of increasing basal synaptic transmission [4]. Furthermore, NO may play a role in astrocytic calcium rises through the NO-G-kinase signaling pathway [52,53]. Conversely, the polyunsaturated fatty acids, arachidonic acid and docosahexaenoic acid, but not eicosapentaenoic acid, blocked intracellular calcium oscillations, inhibited store-operated calcium entry, and reduced the amplitudes of GPCR-calcium responses [54]. The metabolic gliotoxin fluorocitrate suppressed astrocytic intracellular calcium signaling and calcium-dependent glutamate release [50].
Astrocytic intracellular calcium increases can modulate inhibitory synaptic transmission. Photostimulation of astrocytes in the primary visual cortex increased the spontaneous firing of inhibitory neurons in an mGluR-dependent manner [55]. In hippocampal slices, uncaging astrocytic calcium increased the frequency of kainate receptor-depended spontaneous inhibitory postsynaptic potentials (sIPSCs) in nearby interneurons [56] but decreased the amplitude of evoked IPSCs and frequency of miniature IPSCs in an mGluR-dependent manner [57].
Calcium-Induced Gliotransmitter Release
Parpura et al. and others applied bradykinin to cultured astrocytes to stimulate internal calcium elevations, which resulted in astrocytic glutamate and aspartate release into the media [58–60]. Bradykinin also led to NMDA receptor-mediated neuronal calcium elevations in neuron-astrocyte cocultures, but not in neurons cultured alone [60]. Photolysis of caged astrocytic calcium increased astrocyte membrane capacitance [61] and potentiated transmitter release at synapses [49]. Innocenti et al. [62] used an enzyme-linked assay system involving glutamate dehydrogenase, an enzyme that metabolizes glutamate and consequently reduces NAD+ to NADH, to measure extracellular glutamate levels in astrocyte cultures. Stimulation of intracellular calcium produced increased levels of NADH, corresponding to glutamate release. Similarly, mGluR agonist trans-aminocyclopentane-trans-1,3-dicarboxcylic acid (trans-ACPD)-induced release of glutamate evoked increases in extracellular NADH and, in most cells, an increase in whole cell capacitance [63]. Propagation of calcium waves occurred at a rate of 10–30 μm/s and glutamate release underlying NADH detection propagated at an average speed of 26 μm/s [64]. Depletion of internal calcium stores reduced the accumulation of NADH [62]. Other gliotransmitters may be released in a similar mGluR- and calcium-dependent manner including D-serine [65], ATP [66–68], and, in the rat olfactory bulb, GABA [69].
The release of gliotransmitters may play a role in plasticity. D-serine released from astrocytes acts as a coagonist for NMDA receptors; therefore, the degree of astrocytic coverage of neurons may govern the level of activation [70]. Clamping internal astrocytic calcium, depletion of D-serine, or disruption of D-serine release from astrocytes blocked long-term potentiation (LTP) in hippocampal slices from rats [71]. This could be reversed by endogenous application of D-serine or glycine and subsequent binding to the NMDA receptor coagonist sites [71]. An mGluR-mediated rise in intracellular calcium and subsequent ATP release multiplicatively scaled glutamate synapses [68]. This effect occurred quickly but was long lasting. Whisker stimulation induced delayed onset local field potentials (LFPs) in the barrel cortex whereas the combination of whisker stimulation with electrical stimulation of the nucleus basalis of Meynert (NBM) induced surges of intracellular calcium levels, elevated extracellular D-serine levels, and LTP in the cortex [72]. These effects were lost in the IP3R2 knockout mice [72].
Exocytosis From Astrocytes
Astrocytes possess the machinery necessary for exocytosis of gliotransmitters, including the protein machinery necessary to form the soluble N-ethylmaleimide-sensitive factor attachment protein receptor (SNARE) complex [63,73–75]. ATPase, cellubrevin, ras-related protein rab3a, secretory carrier membrane protein, synapsin I, synaptic vesicle glycoprotein 2 A (SV2), synaptotagmin I, synaptobrevin II, synaptophysin, vesicular glutamate transporter 1 (VGLUT1), and VGLUT2 were all expressed in cultured astrocytes [58,63,76–82]. Astrocytic VGLUT3 expression was reported in slices from rats [83]. VGLUT and cellubrevin in astrocytic vesicles were found in close proximity to NMDA receptor-containing neuronal membranes [77]. Glutamate [76] and D-serine [65,84] colocalize with many of these exocytosis markers in cultured astrocytes. Pharmacological stimulation of intracellular calcium led to recruitment of synaptobrevin to the plasma membrane with concomitant disappearance of D-serine, suggesting calcium-dependent exocytosis [84].
The expression of neuronal syntaxin and SNAP-25 protein of the fusion complex have also been reported in cultured astrocytes [85] but their expression is controversial [58,80]. SNAP-23 is an analog of SNAP-25 and has been reported in both astrocyte cell cultures [79,86] and in the rat cerebellum [86]. Astrocytes analyzed immediately after isolation from 11-day-old animals were immunopositive for SNAP-23 and synaptobrevin II, almost entirely devoid of SNAP-25 and synaptophysin, and completely devoid of SV2 (Figs. 6.1 and 6.2) [82]. When cultured for various lengths of time, however, the expression of all proteins were abundant, suggesting that culturing astrocytes can induce the expression of certain proteins [82]. Jeftinija et al. [58] found that astrocyte cultures lacked SNAP-25 immunoreactivity, but pretreatment with BoTx-A (cleaves SNAP-25) and BoTx-C (cleaves syntaxins) decreased baseline and bradykinin-evoked glutamate release. After bradykinin-induced glutamate release in astrocyte cultures, α-latrotoxin stimulated calcium-independentglutamate release from astrocytes [59]. Since α-latrotoxin induces vesicle fusion and calcium-independent release of neurotransmitters from neurons, it suggests that astrocytes may release gliotransmitters in a similar manner. Furthermore, botulinum toxin B (cleaves SNAP-25) and tetanus toxin (cleaves synaptobrevin) decreased synaptobrevin II immunoreactivity and abolished glutamate release in cultured astrocytes [58,87]. The vacuolar type H+-ATPase inhibitor bafilomycin also reduced glutamate release from astrocytes [27,78,87]. The inhibition of glutamate transporters, on the other hand, had no effect on calcium-dependent glutamate release in rat hippocampal cultured astrocytes [87].

Cells were fixed directly after isolation (A, B, J, K) or after culturing for 12 h (C, D, L, M), 1 DIV (E, F, N, O), and 8 DIV (G, H, P, Q). A, C, E, G, distribution of SNAP-25; J, L, N, P, distribution of SNAP-23. B, D, F, H, K, M, O, Q represent the corresponding distribution of EGFP fluorescence, representing astrocytes. Arrows, punctuate immunofluorescence in cellular extensions; arrow heads, punctuate staining in cell bodies. Images were adjusted to improve brightness and contrast. Scale bars: 10 μm. Source: Reproduced with permission from Wilhelm A, Volknandt W, Langer D, Nolte C, Kettenmann H, Zimmermann H. Localization of SNARE proteins and secretory organelle proteins in astrocytes in vitro and in situ. Neurosci Res 2004;48(3):249–57.

Cells were fixed directly after isolation (A, B, J, K) or after culturing for 12 h (C, D, L, M), 1 DIV (E, F, N, O), and 8 DIV (G, H, P, Q). A, C, E, G, distribution of synaptobrevin II; J, L, N, P, distribution of SV2. Note the absence of indirect SV2 immunofluorescence in freshly isolated astrocytes. B, D, F, H, K, M, O, Q represent the corresponding distribution of EGFP fluorescence, representing astrocytes. Arrows, punctuate immunofluorescence in cellular extensions; arrow heads, punctuate staining in cell bodies. Images were adjusted to improve brightness and contrast. Scale bars: 10 μm. Source: Reproduced with permission from Wilhelm A, Volknandt W, Langer D, Nolte C, Kettenmann H, Zimmermann H. Localization of SNARE proteins and secretory organelle proteins in astrocytes in vitro and in situ. Neurosci Res 2004;48(3):249–57.
Both ATP- and glutamate-storing vesicles may be present in astrocytes, but they have distinct properties. For example, ATP release was only partially sensitive to tetanus neurotoxin in cultured astrocytes whereas glutamate release was almost completely impaired [66,81]. In addition, lysosomes found in astrocytes contain ATP and execute calcium-dependent exocytosis of gliotransmitters [88–90]. ATP mediated by P2YR1 is thought to be the major determinant of astrocyte calcium wave propagation (Fig. 6.3) [91]. Blocking lysosome release of ATP prevented the spread of calcium waves [92].

Stimulation of astrocytes results in release of ATP, which binds to P2YRs on neighboring astrocytes and mobilizes intracellular Ca2+. Astrocytic Ca2+ waves are evoked by mechanical or electrical stimulation, agonist exposure, or photolysis of caged Ca2+. Source: Reproduced with permission from Tian GF, Takano T, Lin JH, Wang X, Bekar L, Nedergaard M. Imaging of cortical astrocytes using 2-photon laser scanning microscopy in the intact mouse brain. Adv Drug Deliv Rev 2006;58(7):773–87.
Secretogranin II, a marker for dense-core granules, was detected in the Golgi complex [93] and in a population of dense-core vesicles [93,94] in cultured astrocytes. These vesicles also contained neuropeptide Y (NPY) and underwent botulinum toxin B-sensitive calcium-dependent exocytosis [94]. The activation of mGluRs may lead to calcium-dependent fusion of NPY-containing dense-core granules with cell membrane followed by peptide secretion in astrocytes [95].
Immunogold analysis of rat tissue demonstrated glutamate and D-serine accumulation in synaptic-like microvesicles in the perisynaptic processes of astrocytes [96]. In the rat cerebral cortex, D-serine colocalized with astrocytic processes [79]. The amino acid content of immunoisolated glial vesicles consisted of both glutamate and D-serine but lacked GABA [79]. Furthermore, vesicular D-serine and glutamate uptake is driven by an electrochemical potential generated by V-ATPase, coupled to chloride transport, and is stereoselective [79].
Photostimulation of astrocytes led to transmitter release with a paired pulse index consistent with a presynaptic mechanism of action [49]. Pretreatment with tetanus neurotoxin abolished these flash-induced membrane capacitance increases [61]. Furthermore, reduction of synaptotagmin IV in astrocytes using RNA interference decreased calcium-dependent glutamate release in situ [64]. Electrical stimulation of hippocampal excitatory axons in rat hippocampal slices resulted in AMPA receptor-mediated SICs in oligodendrocyte precursor cells (OPCs) that were both quantal in nature and exhibited rapid kinetics [97]. These characteristics are consistent with exocytosis of glutamate-filled vesicles.
Time lapse confocal microscopy of astrocytes in vitro revealed punctate expression of clear vesicles that were heterogeneous in size [78]. While most of these vesicles remained immobile, subpopulations of highly mobile vesicles capable of fusing with the plasma membrane at astrocyte processes existed [78]. Furthermore, a separate study using cultured hippocampal astrocytes from rats estimated quantal release of only about 10% of total vesicle content during a physiologically stimulated release event via a “kiss-and-run” mechanism [98]. Full-collapse fusion has also been found to occur in astrocytes [99]. Rapid phase exocytosis was sustained almost entirely by vesicles undergoing kiss-and-run fusion whereas slow phase exocytosis was maintained by new vesicles undergoing full-collapse fusion. Intracellular calcium rises occurred near sites of exocytosis and were in strict temporal and spatial correlation with full-collapse fusion events [99] in a manner similar to neuronal exocytosis.
Modulation of Calcium-Dependent Exocytosis
Various chemical mediators have been found to modulate calcium-dependent exocytosis. Endocannabinoids activate CB1 receptors on astrocytes, which leads to phospholipase C-dependent release of intracellular calcium stores and release of glutamate [48,100,101]. This may lead to LTP if accompanied by postsynaptic NO production [100]. Prostaglandins mediate the activation of both AMPA/kainate receptors and mGluRs on astrocytes in acute slice preparations [102]. The application of prostaglandin E2 promoted calcium-dependent glutamate release whereas the inhibition of prostaglandin synthesis prevented the release [102].
Tumor necrosis factor α (TNFα) and its receptor TNF receptor 1 (TNFR1) play a role in the modulation of glutamate secretion from astrocytes. Using both wild-type and TNFα-deficient neurons and glia, Stellwagen and Malenka [103] demonstrated that synaptic scaling in response to activity blockade is mediated by TNFα released from astrocytes. In hemibrain horizontal slices from wild-type mice, TNFα activated astrocyte P2YR1 as well as induced astrocytic calcium elevations and glutamate release [104]. In mice lacking TNFα, however, intracellular calcium elevations could still be obtained, but glutamate release and neuromodulation could not. Cultured astrocytes from TNFα-deficient mice exhibited a defect in functional docking of VGLUT-expressing synaptic-like microvesicles [104]. Activation of the G-protein linked CXCR4 receptor by the chemokine stroma cell-derived factor 1 in hippocampal slices resulted in the rapid release of TNFα and subsequent stimulation of calcium-dependent glutamate exocytosis, a process that was absent in TNFα-deficient mice [105]. Interestingly, reactive microglia amplified this glutamate release.
Controversy Over Calcium-Induced Exocytosis
The above data collectively constitute convincing evidence for the vesicular release of gliotransmitters in response to Gq-linked GPCR-dependent calcium elevations within astrocytes. This release can be sensed by neighboring neurons and is thought to play a role in the modulation of neuronal activity. The calcium-dependent mechanism of gliotransmission, however, has been called into question for several reasons [106]. First, transgenic mice that express a Gq-coupled metabotropic receptor (MrgA1) only in astrocytes were created [107]. Activation of these receptors produced robust, widespread astrocyte calcium increases in acute mouse hippocampal slices but failed to increase neuronal calcium, produce SICs in CA1 pyramidal neurons, or have any effect on excitatory synapse activity [107]. Second, IP3 receptor 2 (IP3R2) knockout mice lack spontaneous Gq-linked GPCR-mediated calcium increases in astrocytes but neuronal Gq GPCR calcium increases remain intact. In these knockout mice, baseline excitatory neuronal synaptic activity and glutamate levels were not altered [108]. Developmental adaptations in these mice cannot be ruled out until conditional knockouts are created [109]. Third, the majority of studies have been conducted in vitro and may not be representative of the true brain state [74,109,110]. One study reported the lack of vesicular glutamate transporter and synaptic protein (VGLUT1, VGLUT2, synapsin 1, synaptotagmin) mRNA in the astrocyte transcriptome in vivo [111]. These proteins were found in neurons. Fourth, experimental design and pharmacological agents used in preparations often have unintended consequences. For example, slice preparations that remove magnesium from the bath relieve the voltage-dependent block on NMDA receptors, but also may initiate spontaneous calcium signaling [112]. “Specific” agonists and antagonists often have inadvertent targets [23,110]. These considerations should be taken into account when interpreting astrocyte calcium signaling studies.
One major problem with studying astrocytic calcium signaling in vivo has been the lack of available tools. Recent developments of new tools that enable real-time monitoring of calcium dynamics, however, may alleviate some of the difficulties. First, genetically encoded calcium indicator (GECI) technology will help visualize thin astrocytic processes with an exceptional signal-to-noise ratio [113]. Second, genetically altered mice, when fully characterized, can prove to be powerful tools [107]. For example, the reporter mouse PC:G5-tdT when crossed with GFAP-Cre drivers can reliably report astrocytic calcium transients [114]. Third, in utero electroporation techniques have allowed for the stable transfection of cells with plasmids featuring the GECI GCaMP [114]. Fourth, excellent calcium imaging has been accomplished using two-photon microscopy after loading of fluorescent indicators in vivo [91]. The creation of better pharmacological, astrocyte-specific tools and the creation of conditional knockout mice combined with powerful imaging strategies will be required to help elucidate the role of astrocyte calcium signaling in healthy and diseased brain.
Astrocyte Calcium Signaling in Epilepsy
Several lines of evidence have suggested that calcium elevations in astrocytes may result in the vesicular release of gliotransmitters, including glutamate and ATP. The role that these calcium spikes and subsequent gliotransmitter release play in epilepsy, however, has not been well studied. Thus far, few human tissue and animal model studies have examined this topic. Existing evidence suggests that dysregulation of calcium channels and increases in astrocytic calcium signaling may contribute to hyperexcitability and subsequent development of seizures [91,115–119].
Human Tissue Studies
Astrocytes cultured from human epileptic foci exhibited spatially and temporally coherent calcium waves [120]. In coculture with neurons, astrocyte cytosolic calcium initially increased in response to high K+, glutamate, or bicuculline and slowly returned to baseline within 10 min (Fig. 6.4) [121]. Calcium imaging combined with electrophysiology revealed spontaneous calcium elevations in cortical and hippocampal slices from biopsies of epileptic patients [122]. These spikes were insensitive to the neuronal activity blocker tetrodotoxin (TTX), abolished by depleting internal calcium stores with thapsigargin, and propagated with the addition of ATP or glutamate. Electrical stimulation led to astrocytic calcium increases and glutamate release, which caused NMDA receptor-dependent SICs in neighboring neurons [122]. These SICs, however, were recorded in the absence of magnesium to maximize NMDA receptor activation, but it is important to remember that the lack of magnesium can have other unintended effects, such as the initiation of spontaneous calcium signaling [112].

Panel (A) illustrates a typical astrocyte loaded with fura-2 in neurobasal medium. Original traces of [Ca2+]c increases after (B) K+ (75 mM, 30 s) or (C) glutamate (500 μM, 30 s) pulse applied as indicated by horizontal bars at the bottom of the traces. Panel (D) shows a representative image of [Ca2+]c induced by 100 μM bicuculline for 15 min from a human neocortical astrocyte after 2 weeks in culture. Source: Reproduced with permission from Cano-Abad MF, Herrera-Peco I, Sola RG, Pastor J, Garcia-Navarrete E, Moro RC, et al. New insights on culture and calcium signalling in neurons and astrocytes from epileptic patients. Int J Dev Neurosci 2011;29(2):121–9.
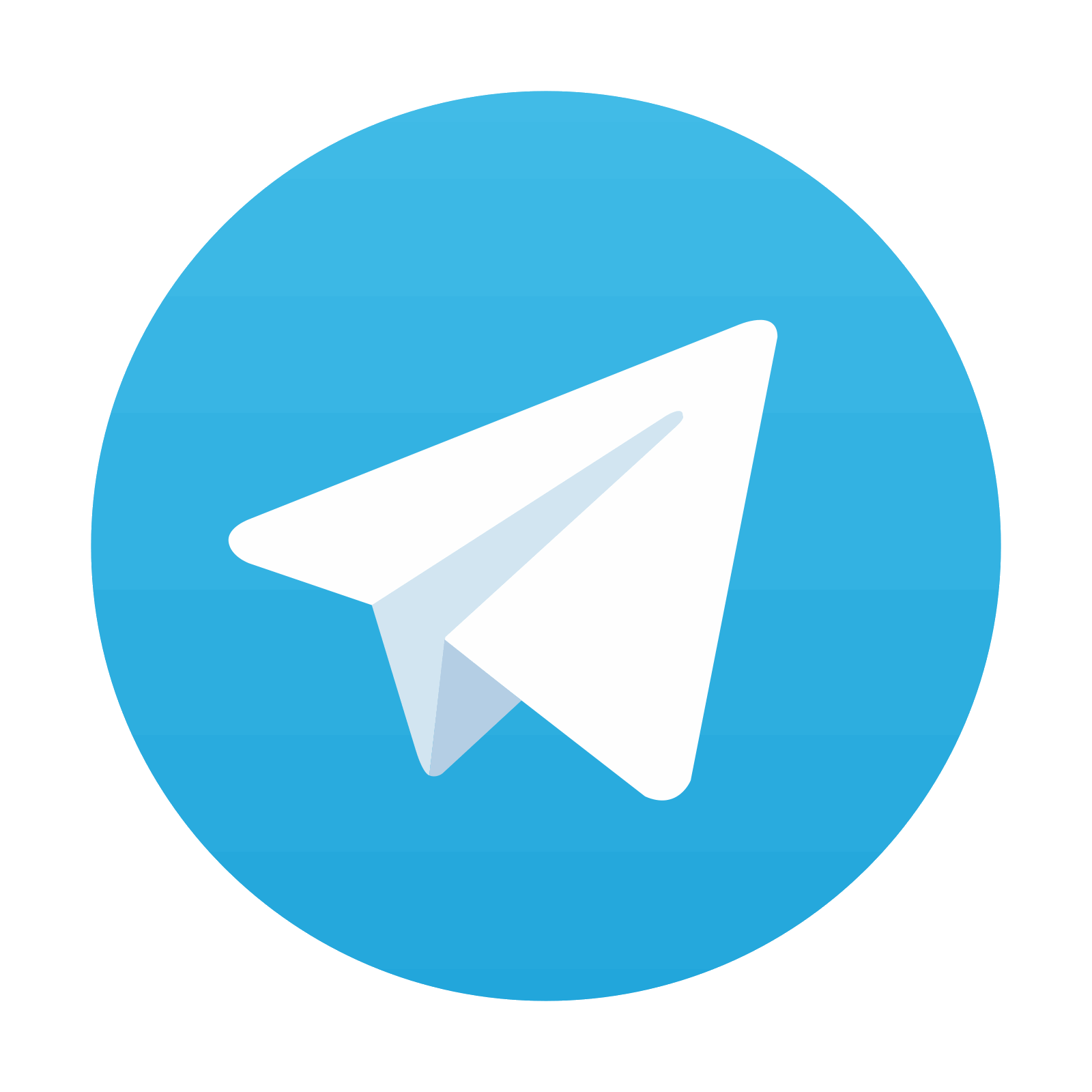
Stay updated, free articles. Join our Telegram channel
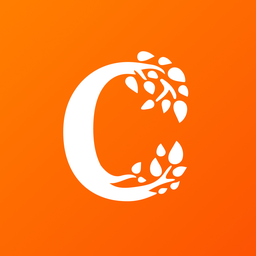
Full access? Get Clinical Tree
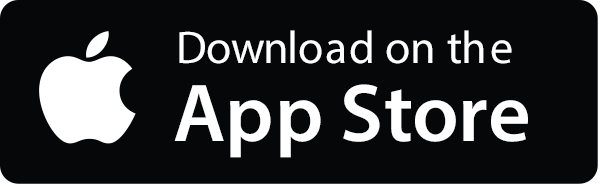
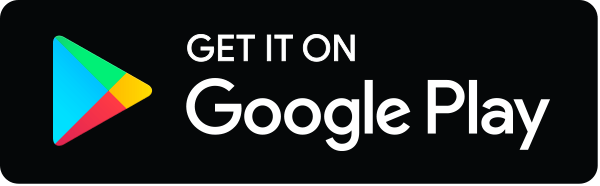
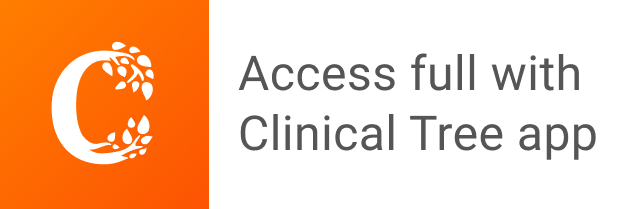