Abstract
Reactive oxygen-induced lipid peroxidation (LP) has been documented to play a critical role in pathophysiology, neurodegeneration, and neurological disability that follows acute traumatic brain injury (TBI) and spinal cord injury (SCI). Consistent with that fact, a number of antioxidant compounds that either scavenges reactive oxygen species or directly inhibit LP have been shown to be protective in preclinical models of TBI and SCI. Although LP is able to damage neural cellular and intracellular membranes, much of the damage is caused by the generation of neurotoxic aldehydic end products derived from peroxidized polyunsaturated fatty acids such as arachidonic acid. Most notably, these reactive compounds, 4-hydroxynonenal (4-HNE) and 2-propenal (acrolein), induce further reactive oxygen species formation and LP. This chapter reviews a relatively new antioxidant strategy involving the pharmacological scavenging of 4-HNE and acrolein referred to as “carbonyl scavenging” that is neuroprotective in SCI and TBI animal models. The prototypes of this class are hydralazine and phenelzine (PZ) that contain hydrazine moieties that can covalently react with the carbonyl function groups of 4-HNE or acrolein thus intercepting them and thus preventing their ability of exacerbate posttraumatic oxidative neurodegeneration.
Keywords
4-Hydroxynonenal, Acrolein, Carbonyl scavenging, Lipid peroxidation, Phenelzine, Traumatic brain injury
Acknowledgments
This work was partially supported by grants from the National Institute of Neurological Disorders and Stroke (NINDS) including 5R01 NS083405 and 5R01 NS084857 and 5T32 NS077889 and with funds from the Kentucky Spinal Cord & Head Injury Research Trust.
Introduction
Oxygen radical-induced membrane lipid peroxidation (LP) is a highly validated secondary injury mechanism that occurs following traumatic brain injury (TBI) that has been firmly established as a major contributor to multiple aspects of TBI pathophysiology due to oxidative damage to neural cell membrane lipids and proteins ( ). A major source of the posttraumatic free radicals that are generated come from leakage of the reactive nitrogen species (RNS) peroxynitrite anion (ONOO − ) which is generated by the diffusion rate-limited reaction of superoxide radicals (O 2 − ) that are generated by a number of sources in the traumatized nervous tissue, and nitric oxide radicals ( NO) produced by both constitutive and inducible nitric oxide synthases. While LP can negatively impact many cellular functions, one of the main consequences of LP-mediated cellular damage is to disrupt mitochondrial functions including mitochondrial respiration/oxidative phosphorylation (ie, ATP generation), cause loss of membrane potential (Δ Ψ ), and impairment of Ca 2+ uptake (ie, Ca 2+ buffering). This ultimately leads to mitochondrial permeability transition (MPT) and dumping of Ca 2+ into the cytoplasm. Collectively, these effects exacerbate intracellular Ca 2+ overload which leads to massive activation of calpains, proteolytic degradation of cytoskeletal and other proteins, and ultimately neurodegeneration schematically outlined in Fig. 13.1 .

TBI represents a major unmet medical need in that despite multiple efforts, no neuroprotective pharmacotherapy has yet been successfully developed to the point of earning FDA approval. Nevertheless, demonstration of neuroprotective effects of various compounds in experimental TBI models strongly suggests that effective clinical neuroprotection in TBI patients should be possible. In view of the nearly successful development of the LP inhibitor tirilazad at least for TBI patients who have traumatic subarachnoid hemorrhage (tSAH) ( ), as well as the repeated demonstration that LP inhibitors can improve neurological recovery and lessen neurodegeneration in rodent TBI paradigms ( ), inhibition of LP remains one of the more promising mechanistic approaches for trying to achieve clinical translation. Furthermore, the fact that much of the LP-related damage is due to the neurotoxic action of aldehydic end products such as 4-HNE and acrolein has offered up a new approach to antagonizing LP-related oxidative damage ( ).
Basics of Free Radical-Induced LP
“Initiation” of LP occurs when a radical species attacks and removes an allylic hydrogen from a polyunsaturated fatty acid (LH) resulting in a radical chain reaction (LH + R → L + RH). In the process, the initiating radical is quenched by receipt of the hydrogen electron from the polyunsaturated fatty acid (eg, arachidonic acid). This, however, converts the latter into a lipid or alkyl radical (L ). This sets the stage for a series of “propagation” reactions which begins when the alkyl radical reacts with a mole of O 2 forming a lipid peroxyl radical (L + O 2 → LOO ). The LOO then reacts with a neighboring LH within the membrane and steals its electron forming a lipid hydroperoxide (LOOH) and a second alkyl radical (LOO + LH → LOOH + L ). Furthermore, the LOOH can be decomposed by interaction with iron. When the LOOH reacts with iron in the ferrous form (Fe 2+ ), this leads to an alkoxyl radical (LO ) or when it reacts with the ferric form (Fe 3+ ) back to LOO , both of which can contribute to further propagation reactions among the free radical susceptible polyunsaturated fatty acids that are heavily enriched in neuronal cell membranes. A number of LP-inhibiting antioxidants have been shown to be neuroprotective in either TBI or stroke models via their ability to limit propagation reactions by scavenging LOO or LO or by decreasing the lipid membrane fluidity which decreases the rate at which lipid radicals can interact with nearby polyunsaturated fatty acids. An example is the LP inhibitor tirilazad whose mechanism includes the ability to react with lipid radicals as well as decreasing membrane fluidity that was able to produce neuroprotective effects in a variety of neurotrauma models ( ). In a phase III clinical trial involving treatment within the first 4 h after moderate or severe TBI, tirilazad was shown to decrease mortality in the subset of patients with tSAH although no overall effect improvement in neurological recovery was apparent in the total population of TBI patients with mixed traumatic pathologies ( ). However, a problem with inhibiting the propagation phase of LP as an antioxidant neuroprotective strategy is that it is associated with a limited therapeutic efficacy window. Moreover, LOO scavengers can only inhibit LP, not reverse it.
Ultimately, the LP process leads to “fragmentation” or “scission” reactions in which the peroxidized polyunsaturated fatty acid breaks down to give rise to the cytotoxic/neurotoxic aldehydic end products 4-hydroxynonenal (4-HNE) and 2-propenal (aka. acrolein) (see Figs. 13.2 and 13.3 ) that contain reactive carbonyl moieties which can covalently bind to basic amino acids such as lysine or histidine as well as sulfhydryl-containing cysteine residues in cell proteins leading to an impairment of protein functions. The covalent chemical reaction of the carbonyl-containing 4-HNE and acrolein with cellular proteins occurs via either Schiff base formation or Michael addition reactions and is illustrated in Fig. 13.3 .


Increase in LP-Derived Aldehydes 4-HNE and Acrolein in Acute Traumatic Spinal Cord or Brain Injury and Association With Mitochondrial Dysfunction
Both 4-HNE– and acrolein–protein adducts are documented to accumulate in the spinal cord tissue during the first hours and days following acute injury ( ). The peak in 4-HNE content occurs at 24 h after injury ( ). A significant portion of the LP-derived 4-HNE–protein adducts are found in mitochondrial proteins ( ). Treatment with the nitroxide antioxidant tempol has been shown to decrease spinal cord 4-HNE content in parallel with an improvement in mitochondrial respiration ( ). The same pattern of accumulation of 4-HNE and depression of mitochondrial respiratory function have been demonstrated in TBI models ( ). However, a decrease in 4-HNE and improved maintenance of mitochondrial respiratory function with the LOO scavenger U83836E ( ) or the mitochondrial permeability transition inhibitors cyclosporine A or NIM811 ( ). These studies show that posttraumatic LP-mediated damage and neurotoxic aldehyde buildup is associated with impairment of mitochondrial respiratory function and that antioxidant protection is able to reverse both. However, it must be cautioned that the damaging effects of LP and accumulation of reactive aldehydes are not specific for mitochondria. While mitochondrial function is believed to be a major target, other cellular organelles, and perhaps the phospholipid-dependent membrane-bound ion pumps in particular, are well known to be susceptible to inactivation by LP and accumulating reactive carbonyls ( ).
have provided a detailed definition of the pathophysiological role of the more potent LP-derived aldehyde acrolein in the context of the acutely injured spinal cord. Initial studies from Shi’s laboratory demonstrated that application of 200 μM acrolein to isolated guinea pig spinal cord caused axonal membrane disruption and conduction loss within 1 h of application ( ). Subsequent studies have shown that the threshold concentration of acrolein for producing impairment of axonal conduction in spinal axons along with increased oxidative stress and mitochondrial dysfunction was only 1 μM ( ). Actual mechanical spinal cord injury (SCI) in a guinea pig compression model leads to progressive accumulation of acrolein and 4-HNE–protein adducts as early as 4 h after injury which then peaked at 24 h ( ). This time course, in the case of the rapid accumulation of 4-HNE, was also shown by Springer and coworkers ( ) and our laboratory using a spinal cord contusion injury model ( ). Moreover, acrolein has been shown to contribute to motor and sensory deficits following SCI ( ).
Comparative Effects of 4-HNE and Acrolein on Brain and Spinal Cord Mitochondria
In studies conducted by our laboratory ( ) and others ( ), it has been repeatedly established that mitochondrial bioenergetic dysfunction and failure is a dominant pathophysiological feature of secondary injury in preclinical models of TBI and SCI. Mitochondrial dysfunction after SCI of TBI is temporally associated with posttraumatic free radical-mediated oxidative damage to mitochondrial proteins and lipids and the accumulation of 4-HNE-modified proteins during the first hours and days after injury. Accumulation of acrolein–protein adducts has also been documented within the injured spinal cord ( ) and is associated with induction of additional oxidative stress and mitochondrial dysfunction ( ).
We have investigated the comparative effects of exposure of Ficoll-gradient isolated noninjured rat brain or spinal cord mitochondria to 4-HNE or acrolein to their effects on mitochondrial respiratory function ( ). Both aldehydes were potent inhibitors of mitochondrial respiration in a dose-dependent manner. 4-HNE significantly compromised spinal cord mitochondrial respiration at a 0.1 μM threshold concentration, whereas 10-fold greater concentrations produced a similar effect in brain. Acrolein was more potent than 4-HNE, significantly decreasing spinal cord and brain mitochondrial respiration at 0.01 and 0.1 μM concentrations, respectively. The results of that study showed that 4-HNE and acrolein can directly and differentially impair spinal cord and brain mitochondrial function and that the targets for the toxic effects of aldehydes appear to mainly include pyruvate dehydrogenase and complex I-associated proteins. Furthermore, they suggested that protein modification by these LP products may directly contribute to posttraumatic mitochondrial damage, with spinal cord mitochondria showing a greater sensitivity than those in brain.
Since the aforementioned studies ( ) involved the application of concentrations of 4-HNE or acrolein as high as 10 μM, one must ask whether these levels are relevant to those estimated to exist in vivo, particularly in injured nervous tissue? Indeed, it has been estimated that acrolein concentrations in the injured spinal cord may reach the range of 100–500 μM ( ).
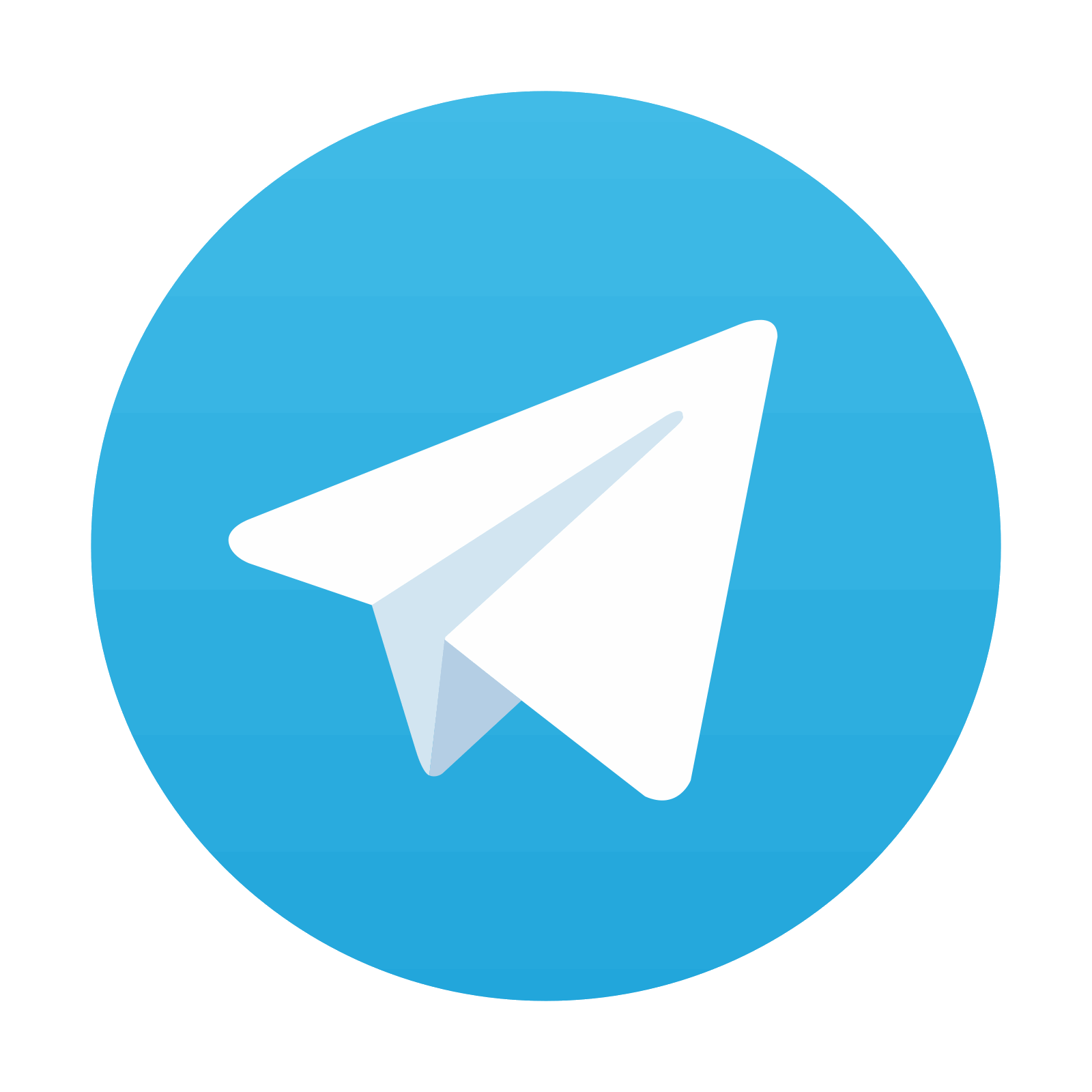
Stay updated, free articles. Join our Telegram channel
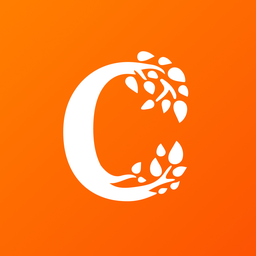
Full access? Get Clinical Tree
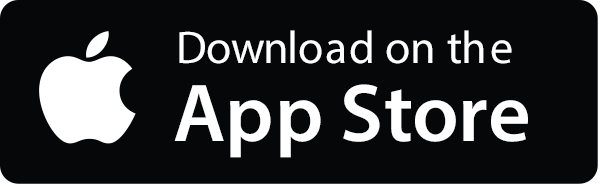
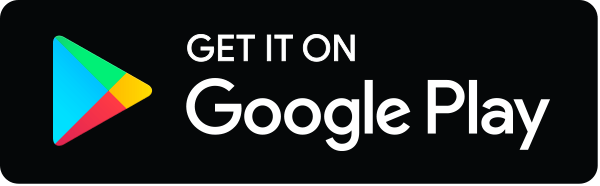