Abstract
Traumatic brain injury (TBI) leads to excess glutamate release from dead and dying neurons, and consequently to secondary neuronal damage weeks and months after initial injury. The GLT-1 (EAAT2 in humans) astrocyte glutamate transporter provides the majority of glutamate clearance in the brain by shunting it into the astrocyte intracellular space. GLT-1 expression, however, is downregulated after TBI, compounding the problem of glutamate toxicity. The β-lactam family of compounds protects against glutamate toxicity by upregulating GLT-1, and among these ceftriaxone is of particular interest owing to high blood–brain barrier permeability. In encouraging preclinical experiments, ceftriaxone shows potential as means to correct disrupted glutamate homeostasis and to mitigate downstream effects of glutamate toxicity. As ceftriaxone is a safe compound that is already in wide clinical use, the translational research with ceftriaxone has a high likelihood to develop into realistic clinical treatment protocols.
Keywords
Ceftriaxone, Glutamate excitotoxicity, Glutamate transport, Neuroprotection, Posttraumatic epilepsy, Traumatic brain injury
Traumatic Brain Injury and Glutamate Excitotoxicity
Traumatic brain injury (TBI) is among the most common causes of morbidity and mortality in the USA across all age groups ( ). Beyond the mechanical injury, TBI triggers a cascade of biochemical events that, while incompletely understood, offers prospects for therapeutic intervention. Among such posttraumatic consequences is disruption of glutamate homeostasis and glutamate excitotoxicity. Progressively increasing levels of extracellular glutamate leaking from dead and dying neurons in the acute and subacute posttraumatic period ( ), coupled with downregulation of glial membrane glutamate transporters in the lesional cortex ( ), lead to the overexcitation of neurons and increased intracellular calcium via calcium-permeable glutamate receptors. Calcium accumulation in turn impacts mitochondrial function and causes increased caspase activation, radical oxygen species (ROS) release, and oxidative injury ( ), eventually leading to cell death. This excitotoxic cycle appears causally related to a range of posttraumatic symptoms, including posttraumatic epilepsy (PTE) as seen by epileptogenic cortical changes that follow TBI ( ).
Long-term posttraumatic sequelae, such as PTE and mnemonic disability are common TBI complications ( ) and are due in large part to neuronal and synaptic dysfunction that is delayed from the time of injury by prolonged time periods ( ). For instance, in human patients and in animal PTE models, epilepsy does not immediately follow TBI. Rather, spontaneous posttraumatic seizures follow a several-week-long seizure-free “latent period” of posttraumatic epileptogenesis ( ). Notably, prospective trials of PTE prophylaxis with common antiepileptic drugs have consistently failed to find an antiepileptogenic benefit ( ) and thus mitigating glutamate excitotoxicity in the latent period is a major area of interest.
Like many aspects of TBI biology, posttraumatic epileptogenesis is incompletely described at the cellular level. However, some aspects appear well-coupled to aberrant posttraumatic glutamate handling. For instance, delayed neuronal apoptosis and exaggerated long-term potentiation of glutamatergic synapses appear to contribute to both PTE and related neurocognitive symptoms. A proximal mechanism for these may be excitotoxic activation of the N -methyl- d -aspartate (NMDA)-type receptor (NMDAR) by free glutamate; and NMDAR-mediated intracellular signaling that leads to transcription genes related to synaptic plasticity and apoptosis appears to be a critical step toward long-term neurodegeneration and abnormal synaptic plasticity that follows TBI ( ).
Glutamate Physiology and Homeostasis
Glutamate is critical for normal brain function ( ). It is synthesized from glutamine in glutamatergic neurons and then stored in presynaptic vesicles. Glutamate release from the vesicles into the synaptic cleft is triggered by calcium influx via voltage-gated ion channels ( ). It is then actively removed from the synapse by astrocytes through membrane transporters ( ), before being converted to glutamine by glutamine synthase and shuttled back to neurons ( Fig. 15.1A ) ( ).

Glutamate acts on both ionotropic (NMDA, alpha-amino-3-hydroxy-5-methylisoxazole-4-propionic acid (AMPA), and kainite) and metabotropic G protein-coupled receptors, prompting intracellular signaling cascades. NMDARs are glutamate-regulated calcium channels that require the binding of glutamate as well as postsynaptic membrane depolarization to remove the magnesium ion blocks the channel at rest, to allow calcium ion influx ( ). Activation of NMDARs and calcium-permeable AMPA receptors (AMPARs) in particular leads to intracellular signaling that promotes potentiation of excitatory synaptic strength, and, if in excess, also triggers apoptotic neuronal death ( ).
Precise control of extracellular glutamate levels is essential for normal synaptic function and for protection against excitotoxicity. Extracellular mechanisms for the enzymatic deactivation of glutamate have not been identified, and so excitatory amino acid transporters (EAAT1–5 in humans) provide the only known endogenous mechanism for rapid glutamate clearance in mammals, by shunting it into the intracellular space ( ).
Glutamate transporter 1 (GLT-1), the rodent analog of human excitatory amino acid 2 (EAAT2), provides 95% of the total glutamate clearance capacity in the mammalian brain ( ). It is a sodium-dependent transmembrane protein that relies on ion gradients across cell membranes generated primarily by the Na/K ATPase as the driving force for glutamate uptake and is present predominantly on glial membranes but is also expressed in oligodendrocytes, presynaptic neuronal terminals, and dendritic spines ( ). GLT-1 (EAAT2) is highly expressed and accounts for as much as 1% of total brain protein ( ).
Astrocytic glutamate transport (hereafter we will use the term GLT-1, as more basic research has been done in rodents than in humans) thus constitutes a powerful mechanism protecting the normal brain from glutamate excitotoxicity. For instance, astrocyte-rich neuronal cultures survive exposure to much higher concentrations of glutamate as compared to astrocyte-poor cultures and both cultures are equally vulnerable to injury after glutamate uptake is blocked ( ). Antisense knockdown of GLT-1 also worsens hippocampal neuronal survival a week after controlled cortical impact (CCI) injury ( ). Loss of glutamate transporters is also associated with neurodegenerative disorders such as amyotrophic lateral sclerosis (ALS) ( ), Alzheimer’s disease ( ), and an experimental model of Huntington’s disease ( ).
Posttraumatic Changes in the Brain: GLT-1, PTE, and Beyond
In the immediate posttraumatic period, Na/K pump failure, membrane swelling, and eventual rupture results in the indiscriminate release of neurotransmitters and ions into the extracellular space. Glutamate excitotoxicity results in rising intracellular calcium that perpetuates neuronal damage ( Fig. 15.1B ) ( ), and the lesion spreads as excitotoxic injury and oxidative stress affect the surrounding tissue long after the initial insult ( ). Yet despite the critical role of acute glutamate-mediated pathophysiologic processes after TBI, there are no clinically available methods to remove excess glutamate in the acute and subacute periods after injury, when glutamate excitotoxicity is likely first to occur.
In the acute (minutes to hours after injury) posttraumatic setting, glutamate transport capacity may not always be beneficial. Glutamate transporters may be a major source of toxic accumulation of extracellular glutamate in a setting of energy failure after injury, when the transporters operate in reverse ( ). The conditions for reversal of glutamate uptake depend on glutamate transporter stoichiometry and transmembrane sodium and potassium concentration gradients that collapse during energy deficiency ( ). Thus, glutamate transporters in areas where TBI causes significant energy failure contribute to glutamate release and subsequent excitotoxicity, while surviving transporters in perilesional regions clear extracellular glutamate and reduce excitotoxicity and neuronal death.
Over a longer period, however, GLT-1 levels are decreased after TBI. GLT-1 expression in the ipsilesional cortex is reduced for up to 72 h after CCI injury in mice, and the reduction in glutamate transport is accompanied by an increase in CSF glutamate concentration ( ). GLT-1 levels are also decreased in the perilesional cortex by close to 50% one week after injury in a rat fluid percussion injury (FPI) model ( ), thus compromising the only known endogenous mechanism for glutamate removal from the extracellular space. The resultant high extracellular glutamate triggers a number of toxic biochemical events. For instance, elevated free glutamate inhibits cystine uptake into astrocytes via the glutamate/cystine antitransporter (SLC7A11, system x c − , or xCT), thus decreasing the amount of cystine available for conversion to cysteine. Cysteine is a critical and rate-limiting precursor for glutathione (GSH), the most important low molecular weight cellular antioxidant ( ). Concurrently, oxidative stress inhibits the activity of EAAT3, which is responsible for approximately 90% of cysteine uptake into neurons ( ), thus limiting the transport of cysteine into neurons. In summary, glutamate excitotoxicity and direct oxidative stress leads to decreased cystine transport into astrocytes and cysteine uptake into neurons, respectively, resulting in decreased GSH levels and further injury.
Receptor and cell population changes may also contribute to continuing secondary damage after TBI. For instance, NMDARs receptors are tetramers of combinations of NR1, NR2A-D, and occasionally NR3A and B subunits. FPI in rat pups leads to a change in the proportion of NR2A and NR2B NMDAR receptor subunit, with a cortical and hippocampal NR2A to NR2B shift which has deleterious functional consequences: NR2B activation results in excessive influx of calcium into the cell and subsequent cell death, and higher numbers of NR2B-containing NMDARs lead to abnormal synaptic plasticity ( ). Thus, targeting NMDARs postinjury is of potential therapeutic value. Indeed, NMDAR blockade following LFPI ameliorates immediate early gene activation in the hippocampus one day after injury ( ). AMPAR expression and function is also impacted by TBI and may lead to excitotoxic injury. Like the NMDARs, AMPARs are also tetramers of protein subunits termed GluR1-4. In the acute phase, GluR1 is upregulated, while NMDAR-mediated GluR2 expression is decreased following TBI ( ). Reduced GluR2 allows for increased calcium permeability of the AMPAR ( ). These changes, along with elevated glutamate levels, ultimately result in increased calcium influx through AMPARs and NR2B NMDARs. The challenge with combating excitotoxicity by inhibiting these receptors, however, is that these cascades are also critical for normal neuronal function when precisely regulated.
Elevated cortical glutamate signaling persists up to 4 weeks after experimental TBI in rodents, the highest being in perilesional tissue, perhaps due to loss of inhibitory interneuron populations which are disproportionately susceptible to delayed damage following TBI ( ). Thus the window of vulnerability to a subsequent insult, and the latent posttraumatic period of epileptogenesis continue for days to months after the initial trauma. The self-perpetuating cycle of neuronal injury and death and compensatory changes causes an imbalance between excitatory and inhibitory processes, ultimately leading to posttraumatic seizures and long-term mnemonic, cognitive, and behavioral changes.
The posttraumatic loss of cortical inhibition can be detected by transcranial magnetic stimulation (TMS), a method for focal cortical stimulation where small stimulating electrical currents in the brain are induced by a strong extracranial magnetic field. With stimulation of the motor cortex by a particular TMS protocol termed paired-pulse TMS (ppTMS), a measure of GABA-mediated cortical paired-pulse inhibition can be obtained in vivo from either humans or rodents with TBI to assess cortical posttraumatic excitatory/inhibitory balance ( ). ppTMS studies in rats show a progressive loss of long interval intracortical inhibition following LFPI in rats ( ), indicative of a growing imbalance between GABA-mediated inhibition and glutamate-mediated excitation or facilitation. This loss ultimately culminates in posttraumatic seizures, weeks after injury ( ).
Ceftriaxone Is a Safe, Widely Used Antibiotic
Ceftriaxone (552 Da) is a third -generation cephalosporin, a family of β-lactam ring containing small molecules that include the penicillins, and exhibit bactericidal activity against a range of gram-positive and gram-negative bacteria. Ceftriaxone inhibits bacterial cell wall synthesis by binding to penicillin-binding proteins, eventually resulting in bacterial lysis, and has been approved by the FDA for use as a safe, broad-spectrum antibiotic for over 30 years. Peak serum ceftriaxone concentrations are obtained 2–3 h after intramuscular administration in humans. It is eliminated unchanged in the urine, and in feces via bile, with a half-life of 6–9 h. After parenteral administration, the drug is widely distributed throughout the body including the brain, especially when the meninges are inflamed, and therefore forms a mainstay of empiric therapy for the disease ( ).
Ceftriaxone Increases GLT-1 Expression and Mitigates Post-TBI Consequences
The prominent role of glutamate excitotoxicity in ALS prompted a search for agents that may enhance glutamate clearance. Accordingly, a screen of over 1000 FDA-approved drugs in 2005 revealed that many β-lactam antibiotics, particularly ceftriaxone which exhibits excellent blood–brain barrier penetration, are potent stimulators of functional GLT-1 (EAAT2) expression in rodents as well as in human cultured astrocytes ( ).
The mechanism by which ceftriaxone regulates GLT-1 (EAAT2) expression is undergoing scrutiny. In isolated human astrocytes, ceftriaxone increases EAAT2 mRNA levels inducing EAAT2 promoter activity via the nuclear factor-κB (NF-κB) signaling pathway. Ceftriaxone activates NF-κB through the degradation of cytoplasmic inhibitor proteins normally bound to NF-κB and the subsequent translocation of liberated NF-κB to the nucleus. Ceftriaxone-mediated EAAT2 induction is then conferred by the NF-κB binding site at the −272 position of the promoter ( ). Marked upregulation of GLT-1 appeared as early as 48 h after the start of ceftriaxone treatment at levels similar to those achieved with meningitic doses of the drug in both p9 rat spinal cord slice cultures and human fetal astrocytes. This upregulation persisted for at least one week in vitro. In vivo ceftriaxone was seen to be effective at meningitic doses in adult rats and led to a threefold increase in brain GLT-1 protein expression 5–7 days after start of treatment ( ).
Ceftriaxone’s neuroprotective capacity in a diverse array of neurologic disease is supported by extensive published data. In TBI rodent models, ceftriaxone improves postinjury outcomes while enhancing glutamate clearance. For instance, ceftriaxone treatment for one week after FPI in rats restores GLT-1 expression in the lesional cortex to near normal levels and reduces posttraumatic astrogliosis 7 days after TBI and also reduces both seizure frequency and duration 12 weeks after injury ( ). A single dose of ceftriaxone after cortical impact injury in rats also prophylaxes against learning and spatial memory deficits and decreases posttraumatic cerebral edema and inflammatory cytokine expression ( ). In these models, ceftriaxone upregulates GLT-1 expression in the initial posttraumatic period, which corresponds to the subacute time course of the initial excitotoxic phase of posttraumatic delayed brain injury and also the initial phases of posttraumatic epileptogenesis ( ).
Beyond TBI, in models of hypoxic and ischemic brain injury ceftriaxone increases GLT-1 expression and improves cell viability in hippocampal slices exposed to intermittent hypoxia ( ), and pretreatment also improves neurological deficit scores and decreases infarct volumes, microglial activation, and IL-1 expression after focal cerebral ischemia ( ). Intracisternal ceftriaxone also reverses the posttraumatic downregulation of GLT-1 after subarachnoid hemorrhage, improves memory functional outcomes, and decreases extracellular glutamate accumulation and hippocampal neuronal death ( ). In addition, ceftriaxone improves behavioral performance in a transgenic Huntington’s disease model ( ) and delays neuronal loss and increases mouse survival in the mouse ALS model, where glutamate excitotoxicity contributes to the disease physiology.
Ceftriaxone Decreases Oxidative Stress Independently of Glutamate Transport
In addition to glutamate-mediated excitotoxicity, oxidative glutamate toxicity may also play a role in posttraumatic secondary neuronal damage ( ) and may be mitigated by ceftriaxone. System <SPAN role=presentation tabIndex=0 id=MathJax-Element-1-Frame class=MathJax style="POSITION: relative" data-mathml='xc−’>?−cxc−
x c −
is an antiporter that takes up extracellular cysteine in exchange for glutamate ( ). This exchange is the rate-limiting step in the synthesis of cysteine for eventual generation of the antioxidant GSH, and this transport system has been linked to numerous CNS processes including protection against oxidative stress ( ), addiction ( ), brain tumor growth ( ), blood–brain barrier function ( ), and neurotransmitter release ( ). Elevated extracellular glutamate inhibits the gradient-driven antiporter ( ), depleting cells of GSH and thus rendering them incapable of neutralizing ROS that are continuously generated by mitochondrial and cellular processes and eventually leading to cell death by oxidative stress. Ceftriaxone increases system <SPAN role=presentation tabIndex=0 id=MathJax-Element-2-Frame class=MathJax style="POSITION: relative" data-mathml='xc−’>?−cxc−
x c −
activity by both increasing extracellular glutamate uptake and increasing the expression of the antiporter via increased nuclear Nrf2 levels, resulting in an increase in intracellular GSH ( ).
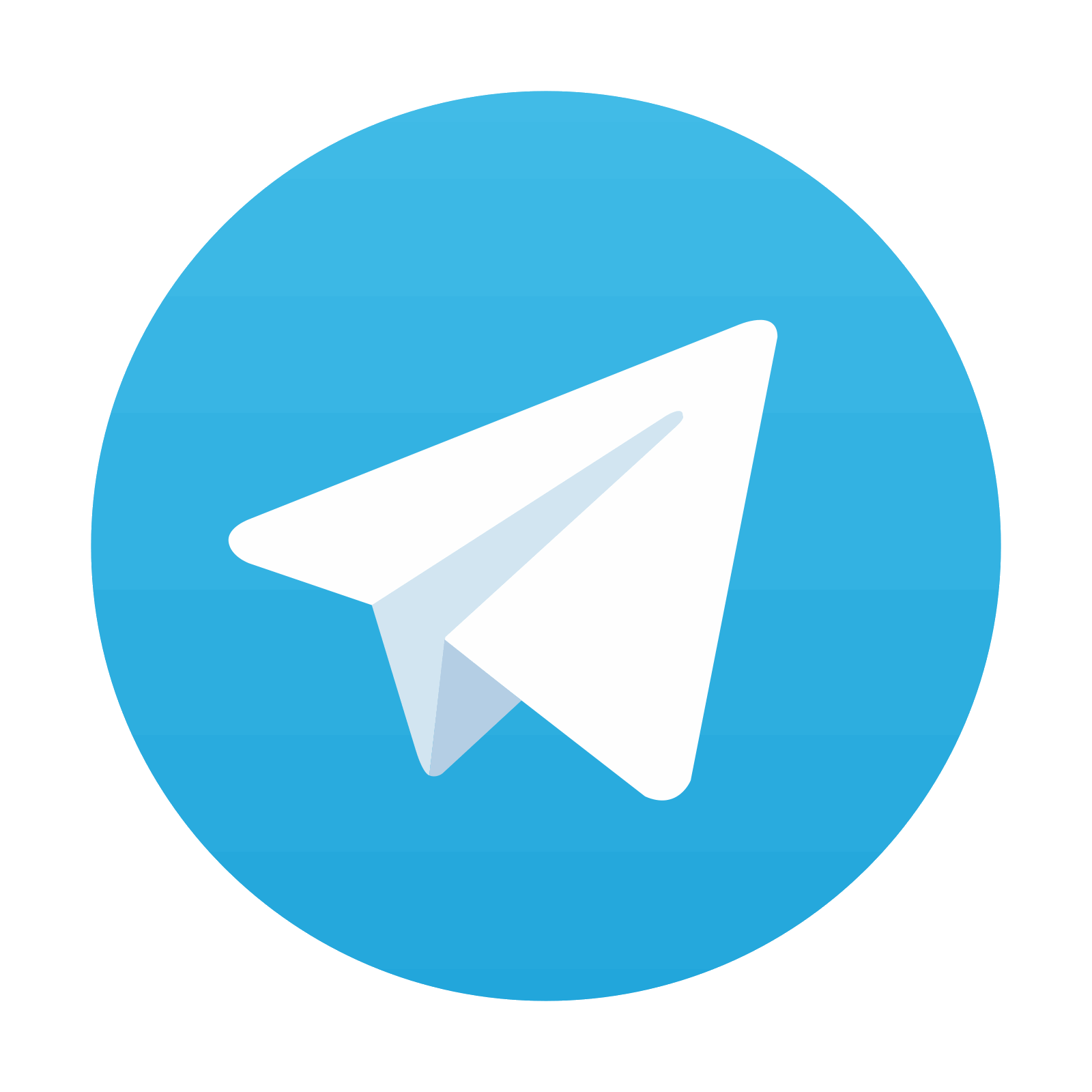
Stay updated, free articles. Join our Telegram channel
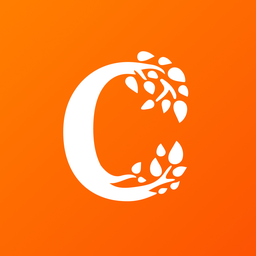
Full access? Get Clinical Tree
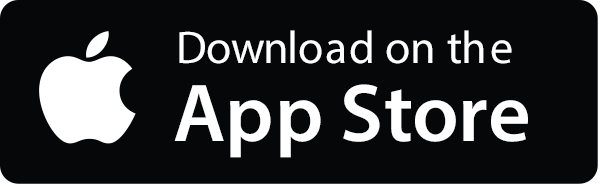
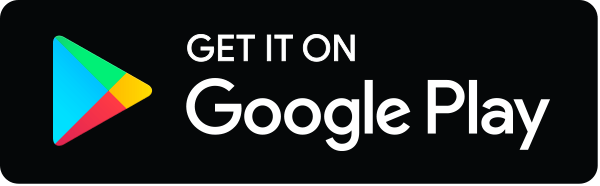