1.1 Introduction
Status epilepticus was originally conceived as the “maximal expression” of epilepsy, being used to describe seizures that “persist for a sufficient length of time or repeated frequently enough to produce a fixed or enduring condition.”1 Although definitions of status epilepticus have evolved with our understanding of the pathophysiology of seizures and epilepsy, the core idea of a self-sustaining epileptic state remains an important part of most definitions of status epilepticus.
Early experiments in primates found that prolonged seizure activity can lead to permanent neuronal damage,2 and subsequent studies have shown that the risk of this rises significantly after 30 minutes of seizure activity.3 Thus, although many clinical studies have moved toward a shorter, operational definition of status epilepticus as seizures lasting longer than 5–10 minutes,4 in order to prioritize the timely administration of antiseizure medication, when considering the risk of longer term brain injury a longer cutoff of 30 minutes remains important.
The importance of status epilepticus is twofold: first, acute episodes of status epilepticus are associated with significant mortality and morbidity in adults (though less so in children); and second, it is hypothesized that episodes of status epilepticus, in particular prolonged febrile seizures (PFS), cause damage to the hippocampus, leading to the later development of mesial temporal sclerosis and temporal lobe epilepsy.
1.1.1 Mortality
Increased short- and long-term mortality have been reported following status epilepticus, however the extent and significance of this risk vary hugely depending on the age and nature of the population studied. Overall it appears that the most significant determinant of short-term mortality is the underlying etiology, with prolonged seizures due to anoxia and cerebrovascular disease having particularly poor prognoses5 (Table 1.1). In both adult and pediatric studies, the majority of cases of fatal status epilepticus are associated with acute symptomatic causes, with much lower mortality from other types of status epilepticus, suggesting that the primary cause of mortality is from the acute neurological insult, rather than the prolonged seizure itself. A number of scoring systems have been developed to predict the outcome of acute status epilepticus, although they have mostly focused on clinical and electroencephalogram (EEG) parameters rather than neuroimaging.6 Magnetic resonance imaging (MRI) is recognized to have an important role in helping to determine the etiology of an episode of status epilepticus,7 but its independent use as a biomarker to determine prognosis has been relatively unexplored to date.
Table 1.1 Mortality of Status Epilepticus
Etiology | Estimated mortality |
---|---|
Anoxia | 60–100% |
Cerebrovascular disease | 20–60% |
Drug overdose/toxicity | 10–20% |
Acute CNS infection | 0–30% |
Idiopathic/cryptogenic | 5–20% |
Alcohol related | <10% |
Prolonged febrile seizures | <2% |
1.1.2 Epileptogenesis
Febrile seizures, that is, epileptic seizures associated with a febrile illness (temperature >38.0C) without evidence of central nervous system (CNS) infection, are a relatively common phenomenon occurring in childhood that will affect around 2–5% of the general population, with a peak incidence at 18 months age.9 Up to 5% of these will be prolonged,10 with durations of >30 minutes.
Mesial temporal sclerosis (MTS) is a structural abnormality that involves not only the hippocampus, but also the entorhinal cortex and the amygdala. Hippocampal sclerosis presents with characteristic radiological (Figure 1.1) and histological (Figure 1.2) appearances. On histological examination there is a loss of neurons in CA1 and CA4 subfields of the hippocampus with atrophy and gliosis. This is reflected in the MRI changes, which show a loss of hippocampal volume on the affected side with an increase in signal intensity on T2-weighted imaging.
Figure 1.1. MRI images of mesial temporal sclerosis With 3D-FLASH MRI sequence (A) showing volume loss of the right hippocampus (arrow), and T2-weighted coronal MRI sequence (B) showing increased intensity of the right hippocampus (arrow).
Figure 1.2. Histological appearance of (A) normal hippocampus and (B) MTS (LFB staining).
MTS is one of the most common lesions found in patients with temporal lobe epilepsy (TLE) and has been described in up to 65% of cases.11 TLE associated with MTS (TLE-MTS) is frequently refractory to medical treatment with antiepileptic medication. It is one of the most common indications for epilepsy surgery. As 30–50% of patients with TLE-MTS have a history of childhood PFS,11, 12 this strong association has led to the long-standing hypothesis that the two are casually related.
According to this hypothesis, subtle hippocampal injury is caused by the initial PFS that subsequently evolves into TLE-MTS. As the mean age of onset of TLE-MTS is 4–16 years,13 this presupposes a prolonged silent period of “epileptogenesis” lasting several years, during which the epilepsy develops, thus potentially providing a window of opportunity for preventative intervention.
As the incidence of PFS is orders of magnitude greater than that of TLE-MTS, some further means of identifying those children at risk of developing TLE-MTS is necessary. The development of appropriate biomarkers to reliably identify children who will develop TLE-MTS following PFS would allow trials of targeted antiepileptogenic treatment to take place using surrogate endpoints without the need for a prolonged 8- to 12-year observation period before judging the outcome.
1.2 The Neuroimaging of Status Epilepticus
In order to develop suitable noninvasive biomarkers for use in status epilepticus, it is necessary to understand the physiological changes occurring during and following status epilepticus and how these are reflected by changes to the neuroimaging appearances, MRI being the general technique of choice.
The uncontrolled, synchronous neuronal discharge occurring during an epileptic seizure leads to significant increases in neuronal energy consumption and metabolism. As a result, blood flow increases, driven by this increasing demand. At some point, as a seizure continues, increases in blood supply become unable to keep up with the increased metabolic demands and the cerebral environment becomes increasingly hypoxic and acidotic with the buildup of lactate as a by-product of anaerobic respiration. At the same time, the recurrent neuronal depolarizations cause increases in the concentration of extracellular glutamate due to saturation of reuptake mechanisms at excitatory synapses and massive influx of calcium into neurons, significantly raising the intracellular concentration. Neuronal injury can therefore occur from both hypoxic and excitotoxic mechanisms, with the risks increasing with seizure duration.
Intractable seizures lasting hours to days have been shown in numerous case studies to lead to progressive cerebral edema, reduced conscious level, and death, with brain atrophy and neuronal cell loss visible at postmortem. The hippocampus is posited to be a particularly vulnerable structure due to its rich connectivity and propensity for burst firing and the vulnerability of its blood supply.14
1.2.1 Human Studies
1.2.1.1 Hippocampal Changes Following Status Epilepticus
There are now a number of neuroimaging studies that have looked systematically at cohorts of children following an episode of convulsive status epilepticus (CSE) and/or PFS (Table 1.2). There is broad agreement from these studies and a number of smaller case series/reports that in the immediate period following status epilepticus there are a number of acute changes visible on MRI suggestive of vasogenic edema within the hippocampus: an increase in hippocampal volume associated with increased signal on T2-weighted imaging and reductions in the apparent diffusion coefficient (ADC). These changes appear to resolve over time, with the majority of children showing apparent normalization of T2 signal and ADC by 48–72 hours post-CSE. Although most of the attention has been on children following PFS, studies that included children with other forms of status epilepticus did not find significant differences in the hippocampal changes reported, suggesting that they may be due to generic effects of a prolonged seizure rather than specific to PFS.15, 16
Table 1.2 Studies of Convulsive Status Epilepticus
Study population | Time of initial MRI | Time of follow-up MRI | Findings | |
---|---|---|---|---|
FEBSTAT study group 201417 | 226 children with PFS | <3 days | 1 year | 22 children showed T2 hyperintensity and increased hippocampal volume on initial MRI, 10 showed MTS at follow-up |
Yoong et al. 201315 | 80 children with SE (33 PFS) | 1–8 weeks | 6 months and 1 year | 15 children showed hippocampal volume loss at 1 year (5 PFS) |
Provenzale et al. 200818 | 11 children with PFS—included within the FEBSTAT population | <3 days | 3–23 months | 7 children showed T2 hyperintensity at initial scan, 5 children showed hippocampal volume loss at follow-up |
Scott et al. 200621 | 23 children with PFS | <5 days | 5.5 months | Increased ADC in children imaged <2 days of CSE |
Scott et al. 2002/200316, 22 | 35 children with CSE (21 PFS) | <5 days | 4–8 months | Increased hippocampal volumes and increased T2 relaxation time in children imaged <2 days of CSE; 5/14 children had significant hippocampal asymmetry at follow-up |
Although hippocampal volumes and T2 changes initially appear to resolve at 48–72 hours, subsequent longitudinal imaging has shown that a proportion of children show ongoing hippocampal volume loss.15, 17, 18
In the largest study, the FEBSTAT group17 found that 11/130 (8.5%) children showed increased signal on T2-weighted imaging at 1-year follow-up; they felt that 10 of these also showed signs of hippocampal atrophy on visual inspection, meeting criteria for MTS. All of the children with MTS had shown hippocampal T2 changes on their initial MRI.
In a separate study, Yoong et al.15 looked at 80 children following status epilepticus; no children met clinical criteria for MTS by the end of follow-up at 1 year, but 15 children showed unilateral or bilateral hippocampal volume reduction over this period. The proportions of children showing volume loss were comparable between children with PFS and those with status epilepticus from other etiologies, suggesting that this phenomenon is not restricted to PFS. Previous work by Scott et al.16 in a smaller cohort of 14 children with PFS had found increased hippocampal asymmetry in 5/14 children at 6-month follow-up, possibly an early sign of developing hippocampal sclerosis.
Taken together, these studies show that there are clearly children who show progressive hippocampal volume loss and develop MTS following PFS and that preexisting MTS as a cause of PFS is very rare. However, this progression appears to occur only in a minority of children (<10%), and at least to the end of existing follow-up periods, relatively few of these children have developed TLE. The long latent period between initial PFS and the later development of TLE-MTS means that it is possible that this remains an underestimate, especially as detecting reduced hippocampal growth rather than an absolute reduction in hippocampal volume is difficult; however, very long-term follow-up of children with status epilepticus19 and epidemiological studies of children with PFS20 are broadly supportive of the hypothesis that only a small proportion of children develop TLE-MTS following PFS and do not provide any evidence of a “hidden epidemic” developing in later adolescence/adulthood.
1.2.1.2 Extrahippocampal Changes Following Status Epilepticus
Studies of patients with established TLE-MTS have shown that the pathology is not limited to the sclerotic hippocampus, but rather that abnormalities frequently extend throughout the temporal lobe and may affect the contralateral hippocampus and other extrahippocampal structures. These are often not detectable from inspection, but become apparent with quantitative MRI analytic techniques. In particular, studies looking at neuronal microstructure using diffusion tensor imaging (DTI) have found significant reductions in white matter integrity in several different white matter tracts bilaterally23 and within bilateral thalami.24
Interestingly, while these appear to correlate with the degree of cognitive deficit and memory impairment seen in TLE-MTS, they do not appear to influence the response to surgical treatment, suggesting an indirect relationship to seizure generation and control.
Relatively few studies have looked at extrahippocampal pathology following status epilepticus. As part of the STEP IN study, Yoong et al. found extensive white matter changes on DTI following PFS.25 These changes were apparent at up to 6 months following PFS but appeared to have resolved by 1 year. The same group of children were also found to show subtle memory impairments,26 although it has not been shown if these are related. This provides further evidence that there may be a longer term impact of PFS other than MTS and that epilepsy may not be the only outcome of interest.
1.2.2 Animal Studies
There are now several animal models of status epilepticus, including models of PFS.27 These models are useful for identifying the pathophysiological mechanisms and potential time course of hippocampal injury and for evaluating the relationship between MRI measures and cognitive outcomes.
There are three main pathophysiological processes associated with hippocampal injury following status epilepticus. The first is related to blood flow. MRI perfusion was carried out during pilocarpine-induced status epilepticus in rats and revealed that blood flow in the hippocampus did not change despite the well-described increase in metabolic demand.28 On the other hand blood flow did increase in the cortex, suggesting that hippocampal homeostatic mechanisms fail during status epilepticus and the ensuing relative hypoperfusion is part of the mechanism that causes hippocampal injury.
The second process is excitotoxicity. Manganese-enhanced MRI (MEMRI) is a tool for exploring whether there has been excess calcium uptake into cells. Manganese competes with calcium, and therefore areas with excessive activation of calcium permeable channels will also allow excessive manganese uptake. The paramagnetic properties of manganese mean that areas with increased manganese will be hyperintense on T1-weighted imaging. Persistent changes in MEMRI imaging are observed from 2 days to 6 weeks after kainic-acid-induced status epilepticus, and the magnitude of these changes inversely correlates with later seizure frequency.29
The third potential mechanism is inflammation. Duffy et al. showed that 24 hours after pilocarpine-induced status epilepticus it is possible to identify areas of inflammation with MRI using antibodies to vascular cell adhesion molecule attached to an iron particle.30 There is evidence for widespread inflammatory changes, although it is not yet known if these changes are predictive of seizure or cognitive outcomes.
Other studies have attempted to identify the time course of MRI changes in the hippocampus using T2-weighted imaging approaches. In the pilocarpine model increases in T2 relaxation time 48 hours after pilocarpine-induced status epilepticus predicts the severity of hippocampal volume reduction. In addition, early studies showed that hyperthermia-induced PFS in rats is associated with the subsequent development of epilepsy in 25–35% of animals.31 MRI at 1 month post-PFS using a 7.0T field strength showed similar changes to those reported after childhood PFS, with increases in T2 relaxation time.32 These were shown to be associated with the degree of neuronal cell loss and interictal EEG activity, but only poorly predictive of epilepsy outcome.
By contrast, a more recent study using the same seizure model scanned rats during the acute phase, immediately following PFS using high-magnetic-field MRI (11.7 T). Significant decreases in T2 relaxation time in the amygdala were found at 2 and 18 hours post-PFS, and these were strongly predictive of the later development of spontaneous seizures. Similar reductions in T2 were obtained using a T2* sequence on a 4.7 T scanner, which may be more replicable in a clinical setting. The T2* change is across much of the brain, including the limbic areas. Interestingly, the reduction in T2* signal was inversely related to behavioral performance on a hippocampal dependent place avoidance task. This suggests that there is a protective mechanism manifest as reductions in T2*, and it is hypothesized that the mechanism is related to efficiency of oxygen extraction in the recovery period following PFS.
1.3 The Use of Biomarkers
As discussed previously, although acute mortality and morbidity are significantly raised following status epilepticus, the role of neuroimaging in predicting these adverse outcomes is limited in the acute situation. At a pragmatic level, tools based on history and examination findings are likely to be more timely and useful in the hospital; although neuroimaging could potentially be used to further refine risk estimates by confirming etiological diagnoses, this remains unexplored and, given the breadth of causes of status epilepticus, is unlikely to result in the identification of a single biomarker.
Similarly, while other aspects of status epilepticus, such as long-term cognitive outcomes, are increasingly being recognized as important, there have been few systematic studies linking these with neuroimaging changes. Data from studies of cognitive impairment in childhood epilepsy,33 as well as from animal studies,34 would suggest that it is the global, extrahippocampal changes across distributed brain networks that are affected and drive these changes. While this provides important insight into the neural substrate of cognitive impairment and its relationship with seizure disorders, there are as yet no obvious biomarkers and the development of predictive models is likely to depend on the combination of a number of different factors.
The main use of biomarkers in status epilepticus at present thus remains the identification of TLE-MTS. It has now clearly been demonstrated that a proportion of children sustain progressive hippocampal damage following a PFS, leading to eventual TLE-MTS. While this is an important finding, it appears to occur in only a small minority of children with PFS; therefore it is difficult to recommend routine treatment with an antiepileptogenic agent, even if such were available, at present. Further investigation as to the efficacy and side-effect profile of any such agent would be necessary, and for this to occur in a realistic time frame will necessarily involve the use of biomarkers. Biomarkers for the development of TLE-MTS might also allow better targeting of any putative antiepileptogenic agent to maximize benefit and minimize any potential harm.
Neuroimaging is noninvasive and has the potential to provide direct information on the pathological changes occurring in the brain following PFS. It is therefore one of the most likely investigations to provide useful clinical biomarkers. Neuroimaging is however not without its costs, especially as most children within this age group will require either a general anesthetic or medical sedation to obtain a good quality MRI scan. It is therefore necessary wherever possible to minimize the imaging burden on individual children and maximize the information gained from the investigation; this may mean that further studies are needed before it can be more widely recommended.
The ideal imaging biomarker for use in children following status epilepticus would reliably identify the children who will go on to develop TLE-MTS. Currently the most promising candidates for this are hippocampal T2 relaxometry and serial hippocampal volumetry.
T2 relaxation time has been shown in both animal models and human studies to be a risk factor for the subsequent development of hippocampal damage and MTS. There remain some uncertainties about the optimal timing of MRI and threshold values for increased risk; the FEBSTAT study stratified using visual assessment and did not quantitate T2 relaxation times. Data from other studies are difficult to interpret: long-term T2 changes were demonstrated by Provenzale et al.,18 whereas Scott et al.16 reported that the initial T2 changes resolved at the 6-month follow-up. Similarly, earlier animal studies showed increased T2 relaxation times following PFS,32 whereas more recent studies using the same model found decreases in T2 relaxation time associated with subsequent epilepsy.35 Much of this could be explained by the use of different time points, magnet strength, and imaging protocols; this serves to underline the fact that T2 changes following status epilepticus are a dynamic and evolving phenomenon and hence the choice of timing and methodology will be critical to determining their predictive value. If the optimal use of T2 imaging can be identified, it has the advantage of requiring only a single time point, and likely one very close to the seizure event to predict outcome.
It makes theoretical sense that early hippocampal volume loss may also be a suitable marker for early injury and the subsequent development of MTS. Progressive loss of hippocampal volume would seem to be a prerequisite for the development of MTS, and longitudinal studies from the FEBSTAT and STEP IN cohorts has now delineated an approximate time scale for this to occur. There are however a number of issues that may make this less suitable as a biomarker in practice: the measurement of hippocampal volume at this age currently relies mainly on manual segmentation, as automated methods have generally been optimized in adult populations and transfer poorly to this age group. Although the development of neonate and infant-specific atlases36 as well as novel machine-learning techniques37 means that progress in this area is likely to be rapid, some caution will need to be exercised in applying novel techniques to potentially abnormal hippocampi as sources of error may not be obvious. An additional problem arises due to hippocampal growth rate during this period of development. Hippocampal growth is expected to be highest within the first 2 years of life,38 and this overlaps with the time of greatest risk for febrile seizures. Detecting an effect on hippocampal volume, whether this be volume loss or a reduction in growth, requires longitudinal imaging at two time points at least. Nevertheless, the time delay required needs to be considered, and whether it would allow the identification of children in a timely enough manner to allow intervention remains unclear.
1.4 Conclusion
The development of a noninvasive biomarker of status epilepticus offers the opportunity to finally attempt to address the neuronal damage and subsequent epileptogenesis that can occur following status epilepticus. We now have plausible candidates for biomarkers for TLE-MTS following PFS, although further development and validation will be required before they are ready for use in a clinical setting or trial.
References

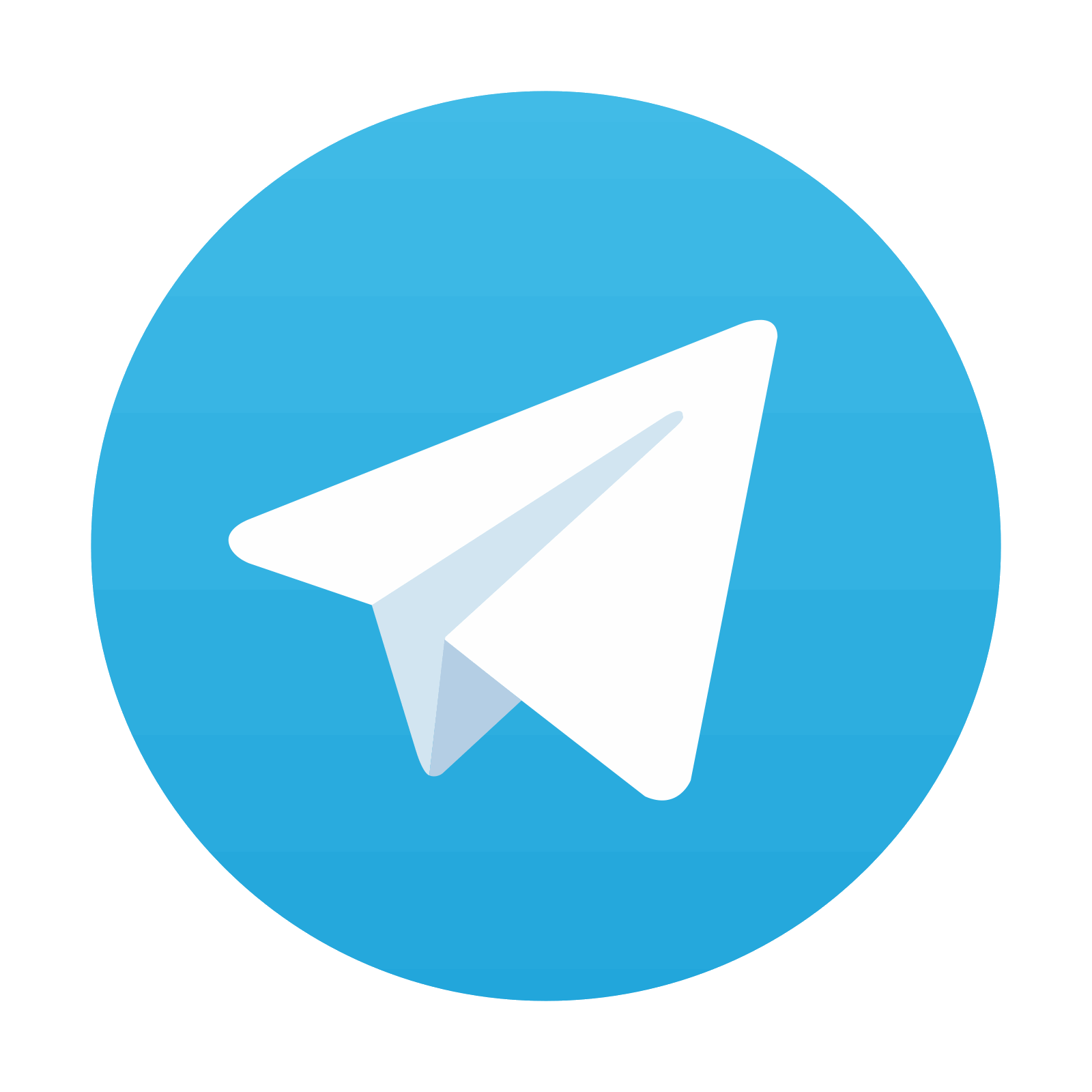
Stay updated, free articles. Join our Telegram channel
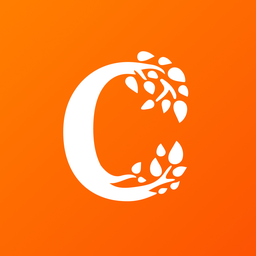
Full access? Get Clinical Tree
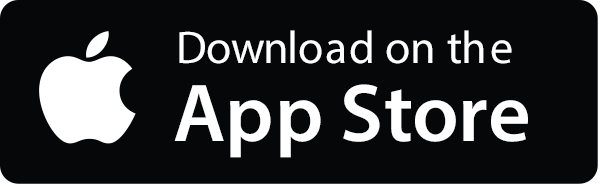
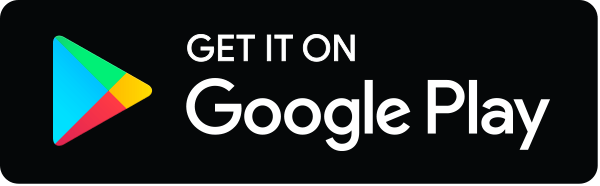
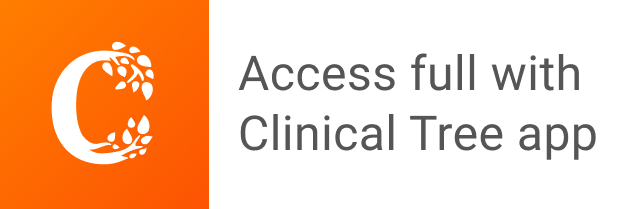