18.1 Introduction
This chapter reviews imaging data on normal brain development, and examines the effect of epilepsy on these systems. FDG-PET, SPECT, and an array of MR imaging provide insights into brain development. These data allow in vivo assessments that recapitulate the elegant anatomic studies of human cortical development1 and myelination.2 This chapter does not review the neurobiology of fetal brain development including cellular division and migration. The process in general is of the establishment of synaptic connections, synaptic pruning, and myelination, a process not “complete” until 25 years although learning, and its underlying biochemical, biophysical, and synaptic processes continue throughout the lifetime.
18.2 Control Data for Normal Brain Development
One challenge has been to obtain data from normal, typically developing “controls.” Infant MRI can be obtained as children sleep, and children above four years can be easily imaged unless significantly developmentally delayed, anxious, hyperactive, or oppositional. One can more readily obtain reliable data with children with developmental and intellectual disabilities after nine years of age.3 As sedation is not warranted, it has become more challenging to obtain data in children three months to four years of age, often obtained when asleep during nighttime studies. There is a large cohort of cross-sectional and longitudinal structural MRI data acquired on 1,500 children for the national NINDS, NIMH, NICHD initiative on 1.5 T scanners, and several other large datasets with children scanned every year for three to four years creating a matrix of small-scale, overlapping, longitudinal data for children ages 4/5 years to 18 years.4–8
For cognitive studies, children can perform tasks such as motor tapping and listening to stories at age three (admittedly the more precocious children and IQs are in the 120s). However, the paradigms must be developmentally and cognitively age appropriate. For example, children younger than seven find it difficult to perform verbal fluency tasks and semantic decision tasks. Younger children tend to have higher IQs; most fMRI development research has mean IQs in the high normal range (e.g., 115).9–20 Thus, there is a slight selection bias for many functional imaging studies.
For infants and young children, passive listening tasks and motor tasks have been conducted when asleep or lightly sedated as deep anesthesia obliterates the blood-oxygen-level-dependent (BOLD) response.21–24 Resting state can be obtained, but it is more difficult to know what occurs during resting state when asleep, lightly sedated, or in the very young, let alone in an older child or an adolescent.
FDG-PET and Xenon SPECT confer radiation exposure, which is, understandably, tightly regulated in patient populations and for clear reasons not permissible to perform in typically developing infants, children, and adolescents. Some “normative” data have been obtained to evaluate children thought to have or be at risk for developmental disorders (e.g., children with port wine birthmark in V1 distribution and suspected Sturge-Weber), or in children with focal abnormalities isolated to one hemisphere with the normal hemisphere used as the control or normal hemisphere (see below).
18.3 Metabolism and Blood Flow
Two FDG-PET studies examined local cerebral metabolic rates for glucose, a measure of cellular energy demand that is primarily synaptic in origin.25, 26 These studies show increasing cortical glucose uptake rapidly increasing to five years, then plateauing, then decreasing to adult levels in late adolescence.25, 26 Cerebral blood flow (CBF) is coupled (generally) to metabolic consumption but is an indirect measure of metabolism. Xenon SPECT studies show a similar pattern.27 These studies are interpreted as showing the growth in cortical thickness, synaptogenesis, and subsequent pruning (Figures 18.1, 18.2). These trends are global, though there are differences in some regions. These regional differences are most pronounced in the first year of life when most glucose uptake is found in motor cortex, less thalamus, and visual cortex, followed by cerebellum. Later in infancy glucose uptake is seen in most other cortical regions to varying degrees. This pattern helps explain why these regions are most vulnerable to perinatal hypoxic ischemia. This primary motor and sensory cortex achieve adult values earlier during development; association cortex achieves adult maturity latest.
Figure 18.1. Synaptic density in auditory (filled circles), calcrine (open circles) and prefrontal cortex (xs) across ages. From Huttenlocher and Dabholkar et al., J Comp Neurol. 1997.
Figure 18.2. A. LCMRGlc across ages ascertained by 18FDG-PET B. rCBF across ages ascertained by Xenon SPECT. Adapted from Chugani et al., Ann Neurol. 1987. Originally published in Chiron et al. Changes in regional cerebral blood flow during brain maturation in children and adolescents. J Nucl Med. 1992;33:696–703.
18.4 Structural Imaging
High-resolution T1 weighted images show general brain development but can also be used to examine sulcation as well as cortical thickness. These investigations have been primarily performed with children aged five years and older. As the cortex is only two millimeters thick on average, high-resolution images are necessary to properly measure changes in the thickness of the cortical ribbon and clearly demarcate it in relation to underlying white matter. T2 images are necessary to assess best maturation of white matter. The main developmental process is thinning of the cortex with maturation (Figure 18.3). Once the cortical mantel is established, the developmental decreases in cortical thickness vary in degree and by regions. There are regional differences in the time line of achieving cortical thickness seen in adults. The primary motor and sensory cortex are the first to mature. The association cortices, frontal and parietal, are the last to achieve adult thickness. The phenomenon of cortical thinning is thought to reflect synaptic pruning.5–8, 28, 29 The consequence of these findings is that efforts to identify regional atrophy or other changes in structural measures caused by, or associated with, epilepsy need to be assessed in the context of normal measures and trajectories of structural development. Between age five years and young adulthood there is little change in brain volume, however there is a decrease in overall gray matter volume due to the cortical thinning described above, and an increase in white matter volume due to myelination described below, presumably reflected in structural and functional connectivity.28, 29
Figure 18.3. Changes in regional cortical thickness with age. From Gogtay et al., PNAS 2004.
Diffusion tensor imaging can be used to identify mean diffusivity and fractional anisotropy (FA). With maturation of myelination there are changes in these measures. These measures can be applied to known long white matter tracts and show the myelination (decreased mean diffusability and increased anisotropy) of the known major matter tracts29–34 (Figure 18.4). The main maturation of these tracts occurs before the age of two, after which there are modest changes, although full association cortex maturation—e.g., in frontal lobes—is not fully mature until the mid-20s (when automobile insurance rates decline).2 As with previous modalities, sensory motor tracts are the first to fully myelinate. The fibers that sustain language mature in the first few years of life. Developmental milestones generally reflect the myelination of these systems, for example, fixing and following in the first month of life, reaching at age four months, and walking at age 12 months. Language processing and expression begins during infancy though main language skills are solidly present by 4–5 years—receptive language followed by expressive language.35–37
Figure 18.4. Fractional anisotropy in white matter tracts across ages (n = 202). From Lebel et al., NeuroImage 2008.
Imaging of infants is particularly challenging as the lack of myelination and high water content makes visualization of gray-white matter boundaries problematic. There are known and well-delineated changes in T1- and T2-weighted images over the first two years of life. Maturation follows myelination, which occurs in a medial to lateral and caudal to rostral pattern. During these early years, myelination is marked by increased T1 W white matter signal as T1 shortens. In contrast, T2 shortens with maturation of myelination reflected in reduced white matter signal. For example, at 12 months the T2 W signal in frontal subcortical white matter is the same as gray matter.4 Therefore, it may be very difficult to identify malformations of cortical development between 3 and 18 months and they may not be well visualized until after 24 months.38 The optimal time to identify malformations of cortical development is less than 3 months and after 24 months. FLAIR imaging and 3D T1 weighted images are the least helpful in this age range (<2 years); rather, T2-weighted sequences in three different axes (or 3D) are the best. Thus, any study that seeks to identify changes over time as a cause or consequence of epilepsy must view observed changes and findings in the context of normal changes in signal that reflect white matter maturation, especially if they are voxel based.
Malformations of cortical development are the most common finding and etiology in children with new-onset seizures and epilepsy in infants (those older than one month but younger than 2–3 years).39, 40 Identification of etiology has prognostic features. The identification of structural abnormalities is associated with high likelihood of continued seizures and increases the risk of developmental arrest associated with epileptic encephalopathy (and may be surgically remediable).
Mesial temporal sclerosis (MTS) is mostly associated with injury before age 5 years. The principal historical cause is febrile seizures (mostly but not always complex or prolonged), followed by encephalitis, meningitis, and other brain injury. Cross-sectional and longitudinal studies and imaging and anatomic studies find evidence of two hits: typically an initial insult often, but not always, accompanied by status epilepticus, and then progressive atrophy presumably reflecting the effect of continued seizures or interictal activity. MTS can be identified in infants but is more commonly found in older patients.41–48 Imaging children with febrile status may identify those with acute increased signal and acute increased size of the hippocampal formation that may be a marker of risk for developing MTS.45, 46 The fundamental findings of MTS such as atrophy and increased signal are the same in children and adults. The FEBSTAT study emphasizes the need not only for control subject imaging but for longitudinal imaging as well. There is evidence that early T2 signal abnormalities, especially those in CA1 and CA3, may place children at risk to develop MTS and mesial temporal lobe epilepsy. More important, a number of children have fallen off their growth curves for normal hippocampal formation development, suggesting the presence of more subtle but perhaps equally important findings.46 Other data suggest the presence of clear MTS and focal cortical dysplasia indicate poor likelihood of long-term seizure control.49
18.5 Functional Imaging: Task Based
Functional MRI (fMRI) using the BOLD image technique has been used to identify and map cognitive networks in adults and children.
18.5.1 Language
Studies of language fMRI show that the networks that sustain language are fundamentally present by age 4 years.9–13, 18, 50–54 They are regionally distinct and lateralized, representing a diffuse and distributed network for language processing that spans both hemispheres. The temporal lobe “receptive” areas in typically developing children reach adult levels of laterality by age 4 to 7 years seem to be firmly established by age 7 years. The frontal “expressive” language areas are also clearly present and lateralized by age 4 years, but do not achieve adult levels of laterality until age 10 years (determined both by laterality index [the strength of laterality of activation] and proportion of those who meet criteria for atypical language dominance)18, 50, 53 (Figure 18.5). Younger children have greater variability in measures or activation laterality (evidence of immaturity) than do adults, and most “atypical” laterality of language dominance is due to activation in the right hemisphere, which appears to diminish with time and is not related to effort or task difficulty.53 Studies of single word processing (several forms of verbal fluency) in children 7 through the late 20s find (1) some brain regions that are unchanged with age, mainly in the distributed language network; (2) other regions that exhibit performance effects (left occipital temporal, scattered right hemisphere, bilateral mesial frontal); and (3) others that show age effects (greater signal intensity seen in adults, compared to children, in specific voxels (not necessarily activated by task in the traditional sense) seen in left premotor/midfrontal gyrus/superior frontal gyrus. In some regions children showed greater signal than adults (e.g., right and left occipital-parietal). These data demonstrate a complex interplay of regional growth and specialization.19, 20
Figure 18.5. Changes in regional laterality indices across ages for Broca’s and Wernicke’s areas during an auditory word definition task compared to reverse speech. From Berl et al., Hum Brain Mapp. 2012.
Epilepsy populations show a high incidence of atypical language dominance, but the expression of hemispheric language dominance needs to be appreciated in the context of normal development. Atypical language BOLD activation laterality is associated with brain injury before age 6 years, either onset of epilepsy or other evidence of brain injury.55–59 Imaging children at onset of epilepsy observes these findings are present at the onset of epilepsy rather than as a cause of epilepsy (the sole exception may be Rasmussen’s encephalitis, where resection of dominant hemisphere after age 8 years typically results in an aphasia that exhibits a gradual albeit incomplete recovery).60 The anterior regions are more amenable to establishing atypical representation until later childhood and are associated with atypical handedness. Also, frontal systems appear to move along with posterior systems but not vice versa. Thus, atypical language reflects persistence of an immature system of language representation.53, 58, 59, 61–63
Atypical language processing occurs in homologues of the left regions classically described as sustaining language functions54, 62–65 and at the margins of language areas. There is a limited capacity for plasticity, which is constrained anatomically, substantiating structure and function relationships.64, 65 Other data-driven methods confirm atypical language processing confined to homologous regions, but also find different patterns and emphasis of left hemispheric activation for those who are left dominant for language. These patterns likely reflect emphasis on different strategies to perform the language task, those strategies appear to involve executive function networks.54 The interaction between language and working memory networks provides a transition to the developmental expression of working memory and executive function networks.
18.5.2 Executive Function/Working Memory
Executive functions, and working memory in particular, are a set of skills required for goal-directed behavior. Working memory is the ability to keep information in mind. These skills are critical for learning and are associated with higher order cognitive skills. The neural network that underlies these skills involves several regions that span the frontal lobe, parietal lobe, and cerebellum. In addition, lateral prefrontal cortex (PFC) is a lynchpin region found across animal and human studies (for review, see D’Esposito66); however, the PFC is not fully developed until late adulthood. As such, several studies have found differences between children and adults on working memory paradigms using fMRI.17, 67–69 In general, adults engage a wider range of regions than children. Although relatively small (n < 35 children), fMRI studies of typically developing children suggest that the developmental differences in working memory networks likely reflect different strategies.68, 70, 71 In one study, authors propose a developmental shift from the early maturing premotor/striatal/parietal/cerebellar networks in children to ventral prefrontal/inferior temporal networks in adults.70 Other studies noted that children do not engage as many regions in response to increasing working memory load68, 69 and the cerebellum in particular is not as active in children.68 In a longitudinal study, authors propose that the hippocampus is recruited in younger adolescents but not in young adults.71 These differences may be related to methodological variances among the studies including the tasks used and ages included.
Complementary to functional studies, structural MR studies document white matter developmental changes in children. General findings are that (1) large changes occur in children under age 3 years but continue at a steady rate into early adulthood; (2) trajectories of change differ by region; and (3) changes correlate with cognitive development.72–76 Studies with children show modest correlations between diffusion parameters in the superior longitudinal fasciculus (SLF) and working memory skills in children.77, 78 Ongoing and future studies are examining different diffusion acquisitions and metrics that may be more sensitive to the relationship between white matter structure and neuropsychological performance.
Overall findings in patients with epilepsy show that (1) there are decreases in FA and increases in MD often, but not consistently, in frontal white matter and corpus callosum; (2) differences extend beyond the location of seizure focus; and (3) parameters are often associated with neuropsychological performance. However, limitations of current studies include small sample sizes and heterogeneous patients groups across and within studies. In addition, very few studies confirm findings of other studies because regions that are significantly different are variable, which are in part due to differences in analytic approach (some target specific a priori regions of interest (ROIs) vs. whole-brain analysis, TBSS vs. extracting mean FA from an ROI, etc.). A brief summary of findings from diffusion studies in epilepsy populations follows.
18.5.3 General Studies Examining Differences between Controls and Epilepsy Groups
Twenty children imaged 5–6 years after initial diagnosis with idiopathic (mixed focal and generalized) epilepsy, 9 of whom still had active epilepsy, were compared to 29 controls using FSL tract-based spatial statistics. The investigation observed reduced FA and increased MD, for the active group only mainly in the fornix, internal capsule; corpus callosum, superior corona radiata, and superior fronto-occipital fasciculus.79 In another study, 30 children with FLE, assessed with MRTrix, showed reduced FA in the cognitively impaired epilepsy group in posterior regions.80 Fifteen children with Sturge-Weber Syndrome showed decreased FA in ipsilateral prefrontal white matter that also correlated with cognition.81 Eight children with temporal lobe epilepsy showed lower FA in splenium of corpus callosum and internal capsule in addition to correlation with age of onset.82 In eight children with temporal-lobe epilepsy (TLE), diffusivity but not FA differences was observed in temporal lobe and cingulate gyrus.83 Mixed white matter abnormalities were identified in all of 24 patients with focal epilepsy and were concordant for some patients with magnetoencephalography (MEG) findings.84 In the largest study to date, encompassing 40 and 45 children with nonlesional epilepsy, standard FA and MD measurements, as well as graph theoretical analyses, demonstrated white matter differences in frontal, temporal, parietal, and occipital regions, in both FLE and TLE groups, regardless of seizure focus laterality. FA in several right hemisphere regions was associated with neuropsychological performance and right temporal FA correlated with seizure onset.85 The graph theory analysis found more areas of abnormality in the FLE group but no correlation with IQ or seizure onset.86
18.5.4 Studies Related to Language and/or Executive Functioning
In a study of 12 intractable epilepsy patients the FA was decreased in the SLF II, which connects parietal and PFC and is involved in attention and other frontal lobe functions.87 A study of 19 children with idiopathic epilepsy described reduced FA in the posterior corpus callosum and cingulum. A study of 23 children with intractable focal epilepsy being considered for surgery found reduced FA in several white matter tracts including inferior fronto-occipital (IFO), inferior longitudinal fasciculus (ILF), and the SLF.88 In 33 patients with epilepsy and focal cortical dysplasia, diffusion parameters (FA and apparent diffusion coefficient) of left language-related tracts (uncinate, arcuate fasciculus, IFO fasciculus) predicted language impairment.89 Some studies have attempted to use diffusion to determine language dominance by examining the arcuate fasciculus in particular with mixed results in small sample sizes (n = 6 and 13, respectively).90, 91
18.5.5 Memory
Studies of memory functioning in typically developing children using neuroimaging techniques are sparse. Verbal memory in typically developing children (age 7–19) is related to left hippocampal and basal ganglia activation, which declines as age increases.92 Overall, mesial temporal lobe activation may decrease across typical development, while prefrontal cortex activity increases.93, 94 Groups of typically developing children across the age span (8-year-olds, 10- to 11-year-olds, and 14-year-olds to young adults) demonstrate functional changes across development with the hippocampus and parahippocampal gyrus becoming increasingly more specialized for detailed recollection.95 Functional developmental changes in regions of the hippocampus have also been found; episodic recall is associated with activity in the posterior hippocampus in TD children, and in the anterior hippocampus in TD adults.96 Indirect high frequency (HF) activation in children performing a language word definition task are more bilaterally represented in typically developing children and children with left focal epilepsy than in adult controls and patients with left focal epilepsy where HF activation generally follow language dominance.97 These data suggest that material specificity may not be established until adolescence and may explain better postoperative memory performance in children compared to adults.98, 99 In sum, across development mesial temporal lobe activation may decrease, while PFC activity increases and the hippocampus demonstrates functional developmental changes across the longitudinal axis (posterior to anterior).
18.6 Functional and Structural Connectivity
Independent component analysis and principal component analysis of resting-state data reveal several distinct distributed networks representing identifiable cognitive systems. The networks include those involved in executive function and cognitive control.100 Functional connectivity examines the strength of connections based on signal fluctuations between cortical regions. These connections may be adjacent (small world) or more distant (large world). Recent developmental studies of resting-state networks reveal that functional brain development is characterized by a reconfiguration of the balance between long-range and short-range cortical connectivity. Studies with infants show the presence of motor and sensory networks but less reliable identification of frontal-parietal networks.101–103 Between 7 and 9 years, “small worldness” of the whole-brain network is manifested, but the strength of connectivity of the midline frontal-parietal network remains weak but increases with age thereafter.104–107 Application of graph-theoretical methods to analysis of large-scale cortical networks demonstrates that individual network nodes are incorporated into different networks with increasing age from childhood to adulthood, suggesting reorganization rather than strengthening of the same networks with development.104, 108 Further, correlations among nodes closer in distance (segregation) weaken with age, whereas those among nodes further away strengthen with age (integration), and the children have stronger and more subcortical-cortical connections while cortical-cortical connections are more prominent in young adults.109 As regards intrahemispheric and interhemispheric connectivity during childhood development, there are data to suggest intrahemispheric connectivity develops before intrahemispheric connectivity97, 101, 110 and anterior to posterior connections develop last.106
Most studies of connectivity show that structural and functional connectivity is less complex and strong in epilepsy populations compared to controls in focal epilepsy111–118 and generalized epilepsy.118–122 The graph metrics are simpler, which may be common to many developmental and other brain-based disorders. Epilepsy, like most central nervous system and developmental disorders, is associated with simpler, less complex network topology (in other words simpler organizational patterns). What remains unclear is if this is an active process or effectively represents a developmental dysmaturity. The observations may reflect age of insult or epilepsy onset rather than effects of disease on a mature system.
Data for language systems in connectivity in language networks show that there is greater inter- than intrahemispheric connectivity with homologous regions that reverses with age. As children age, intrahemispheric connectivity becomes stronger.97 Also, there are age-related changes in connectivity in the dorsal stream (subserved by the arcuate fasciculus and superior longitudinal fasciculus) and the ventral stream (extreme capsule, uncinate, and inferior longitudinal fasciculus). Stronger connectivity measures are associated with better language abilities. Children with focal epilepsy exhibit particular vulnerability to maturation of the ventral stream. Furthermore, for children with epilepsy, decreased connectivity in the ventral stream is related to poorer performance on language measures.123
There are few studies of EEG fMRI activity and connectivity in children. Studies in childhood absence show a distributed network that is regionally specific involving thalamus and some cortical regions. In addition, the time course can be delineated and relate to the spike or after going slow wave. Studies in adults reveal the remote effects of spike activity of BOLD response.124 Most pediatric studies have been conducted in children with generalized epilepsy, e.g., absence, or in young adults with a history of a childhood epilepsy syndrome. These findings vary in activation and deactivation based in spike or slow wave activity.125, 126 Studies of spike foci in patients with focal epilepsy and rapid generalization and some patients with Lennox-Gastaut syndrome, or with patients with frontal lobe epilepsy with rapid secondarily generalization that share some features of Lennox-Gastaut syndrome, indicate that frontal foci show connectivity to the broadly defined attention network.127–129 These data suggest that some epileptiform activity, depending on location, taps into the distributed networks into which that region is linked that relate to the basic cognitive systems identified in early adult connectivity work. They highlight the effect of epilepsy on distributed networks, and may account for some of the cognitive impairments in patients with epilepsy.
18.7 Conclusions
Brain maturation may be imaged in different modalities. As synaptic connections are formed and pruned, there is regional growth and shrinkage of the cortical ribbon linked to the maturation of the long matter tracts that connect adjacent and far-reaching brain areas. The changes in synaptic density may be assessed with measures of blood flow and cerebral metabolism. Finally, the numerous networks that sustain essential brain and cognitive functions may be assessed by fMRI tasks that show their maturation and perturbations in response to epilepsy. Connectivity analysis and graph theory provide additional means to assess the local and remote functional and white matter connections. These factors need to be considered when evaluating the effect of epilepsy or its cause on brain structure and function at the time of onset and over time.
References




























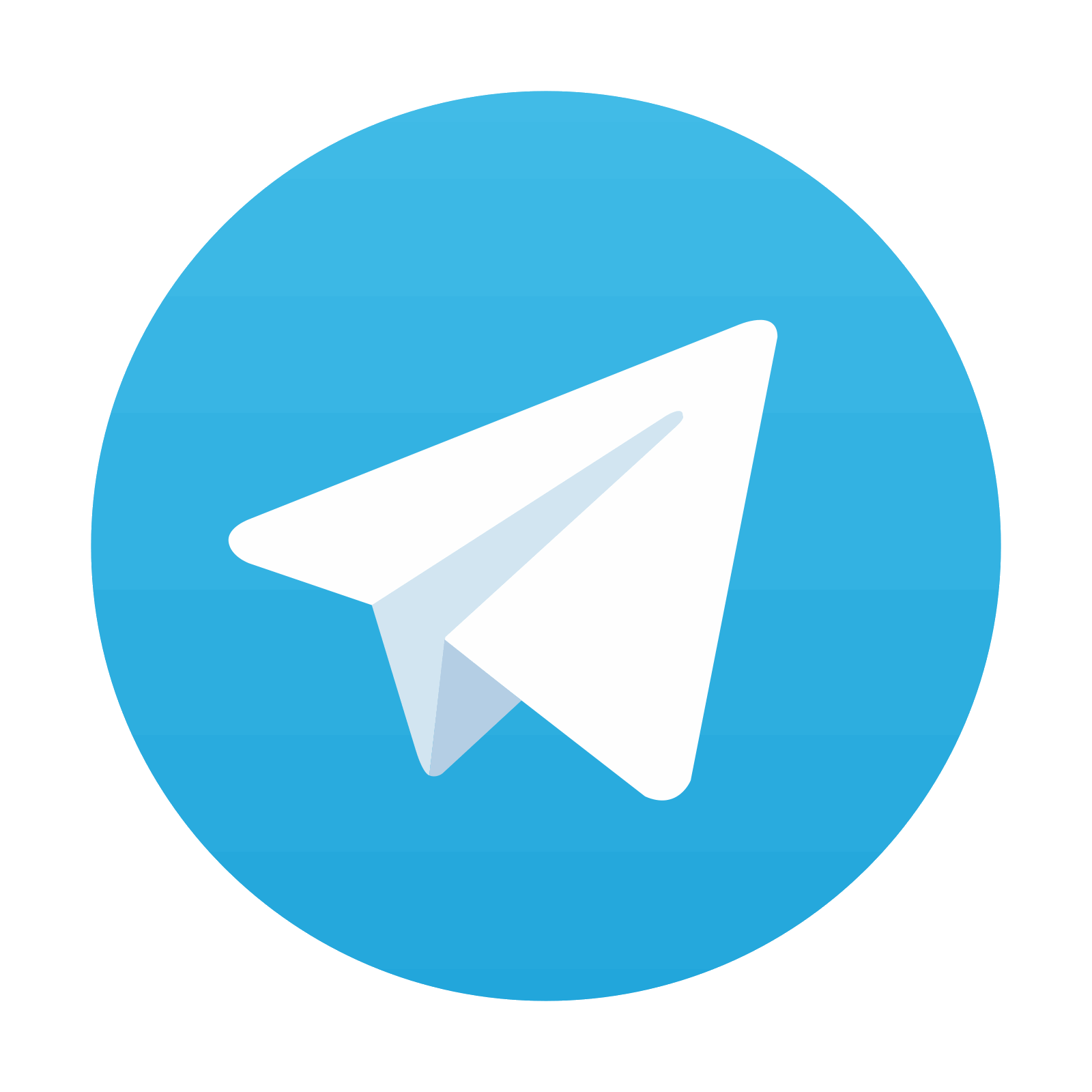
Stay updated, free articles. Join our Telegram channel
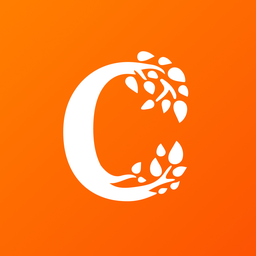
Full access? Get Clinical Tree
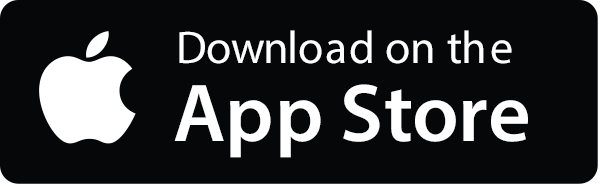
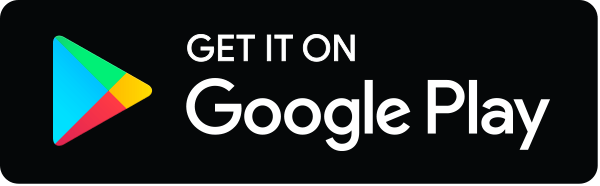
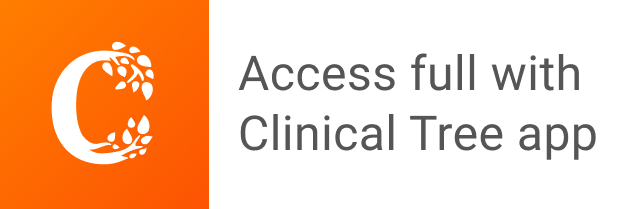