9.1 Introduction
Abnormalities of brain energy metabolism and neuroinflammation are both key aspects of the pathophysiology of epilepsy.1–3 Both metabolic and inflammatory changes in the epileptic brain can be studied by in vivo molecular imaging. Historically, mapping of cerebral glucose metabolism was the first clinical application of positron emission tomography (PET) in the evaluation of epilepsy. Initial studies with 2-deoxy-2[18F]fluoro-D-glucose (FDG) PET demonstrated localized glucose metabolic changes in the form of interictal hypo- and ictal hypermetabolism in patients with focal epilepsy.4, 5 Since then, FDG-PET became a mainstream imaging modality in the presurgical evaluation of patients with medically refractory epilepsy. Subsequently, interest in neuroimaging of epilepsy-associated neuroinflammation emerged following early studies showing increased peripheral benzodiazepine bindings sites, localized on activated microglia, in resected human epileptic tissue.6, 7 Interest in epilepsy-associated neuroinflammation has further increased during the last decade, after the widespread recognition of the pivotal role of neuroinflammatory processes in the pathophysiology of common forms of human epileptogenesis.1, 8, 9
Most PET studies of human epilepsies included patients with medically refractory seizures. These studies provided a great amount of information on chronic metabolic abnormalities in long-standing epilepsy. However, in this patient population, it is nearly impossible to differentiate between changes directly related to epileptogenesis vs. those caused by an underlying process (lesion) or secondary brain tissue damage. Alternative approaches include the study of human epileptogenesis before or shortly after the onset of clinical seizures and imaging studies of epileptogenesis in animal models using small-animal scanners.10
The term “epileptogenesis” refers to a number of molecular, cellular, and brain structural changes, triggered by a cerebral insult, which leads to the first spontaneous seizure(s). The underlying processes of epileptogenesis include, among others, neurodegeneration, gliosis, blood-brain barrier damage, and neuroinflammation.11 These molecular and cellular changes may persist well beyond the initial clinical seizure. Therefore, neuroimaging studies in new- or recent-onset epilepsies may provide a valuable insight in the processes of epileptogenesis. The period of epileptogenesis also provides a critical therapeutic window to intervene with the intent to prevent (or delay) the development of clinical seizures and/or prevent progression of epilepsy and secondary brain damage. There is intense research to understand details of molecular mechanisms of pre- and postclinical stages of epileptogenesis. A critical component of this effort is to identify specific biomarkers to predict development of epilepsy after an insult, identify the severity and extent of brain tissue capable of generating spontaneous seizures, measure progression, and evaluate potential therapeutic interventions.12 For potential epilepsy biomarkers, molecular imaging is a particularly attractive tool to study specific molecular processes in various brain regions in vivo.
In the next sections, we summarize some key molecular imaging studies aiming at mapping brain metabolic and neuroinflammatory processes in both animal seizure models and human epilepsies, focusing on key imaging findings in early and late stages of epilepsy.
9.2 Imaging Markers of Glucose Metabolism in Animal Models of Epileptogenesis
The increasing availability and use of small animal PET scanners in the last 10 years allowed the study of the temporospatial evolution of global and regional brain glucose metabolism in experimental models of epileptogenesis. The most commonly studied models employ pilocarpine or kainic acid to induce status epilepticus (SE). In these models, SE is followed by a latent period before development of spontaneous seizures in a subset of animals. In the early phase (within 1–2 days) after SE, longitudinal FDG-PET imaging can demonstrate a global decrease of cerebral glucose utilization, with maximum decreases in the limbic structures and less robust or minimal decrease in the cortex.13, 14 This is followed by a metabolic recovery in most structures except the hippocampus and thalamus, which remain hypometabolic in the chronic phase (Figure 9.1). Severe hypometabolism in the entorhinal cortex was followed by development of spontaneous seizures, suggesting a critical role of the entorhinal cortex in the early stages of temporal lobe epilepsy (TLE).13 Similarly, widespread brain glucose hypometabolism was reported after kainic acid-induced SE.15 In this model, the hippocampal and thalamic/hypothalamic regions showed a persistent reduction in glucose metabolism, while FDG uptake in the amygdala/entorhinal cortex and motor/somatosensory cortices transiently returned to control levels one week following SE and decreased again at later time points. While hippocampal volumes decrease in the silent and chronic period of epileptogenesis, no correlation was found between the degree of the hypometabolism on FDG-PET and the severity of MRI-detected hippocampal atrophy or CA1 pyramidal cell loss.14, 15
Figure 9.1. MRI coregistered micro-PET images (six selected coronal slices) in the different phases of epileptogenesis in rat brain. Status epilepticus was induced by pilocarpine. The glucose utilization in most brain structures, especially in limbic structures, decreased in the early and latent phases and returned to baseline levels in the chronic phase, with the exception of the hippocampus and thalamus. In the pons and the cerebellum, the glucose utilization remained at baseline levels in all phases. The numbered brain regions are as follows: (1) prefrontal cortex, (2) sensorimotor cortex, (3) striatum, (4) piriform cortex, (5) hippocampus, (6) thalamus, (7) visual cortex, (8) auditory cortex, (9) entorhinal cortex, (10) pons, and (11) cerebellum.
A recent study has also provided data on early metabolic changes after traumatic brain injury in rats.16 In this model, about half of the animals developed spontaneous recurring seizures or epileptic discharges during a 6-month follow-up period. Only a few subtle MRI and FDG-PET abnormalities were predictive of posttraumatic epilepsy. Specifically, a multivariate logistic regression model that incorporated serial PET parameters from the ipsilateral hippocampus predicted the outcome correctly. However, the relevance of these findings for human posttraumatic epilepsy remains to be demonstrated.
Altogether, the animal studies indicate that widespread cerebral hypometabolism is an early metabolic hallmark during SE-induced epileptogenesis, and persistent hippocampal hypometabolism is not simply due to pyramidal cell loss or structural atrophy. While increased metabolism is usually not observed, permanent hypometabolism develops in specific structures (hippocampus, entorhinal cortex, and thalamus) that commonly show focal hypometabolism (and also atrophy) in human TLE.17, 18 Whether limbic hypometabolism is also a common feature of the early stages of human epileptogenesis, where initial SE does not play a significant role in most cases, remains to be clarified.
9.3 Imaging Brain Metabolism in Human Epilepsy
9.3.1 Glucose Metabolic Changes in High-Risk Patients
Early stages of human epileptogenesis can be studied in patients with an epileptogenic brain insult before the onset of the first clinical seizure(s). The overall risk of epilepsy after common brain insults in adults is modest; for example, only up to 5% of stroke patients and around 10% of patients with traumatic brain injury go on to develop epilepsy.19–21 Considering the relatively low epilepsy risk, these lesions warrant a widely available, easy-to-obtain biomarker to identify the subset of patients who are at highest risk for development of epilepsy. While MRI is routinely performed in these patient populations, widespread PET studies are not feasible. For application of PET imaging, a more realistic goal is to identify specific patient subgroups with a very high epilepsy risk, such as children with neurocutaneous disorders, including Sturge-Weber syndrome (SWS) and tuberous sclerosis complex (TSC). These disorders commonly manifest in infancy due to characteristic skin lesions, which typically precede development of clinical seizures. The overall risk of developing epilepsy is around 80% or higher in both diseases. In the vast majority of affected children, seizures start within the first two years of life. SWS is most commonly associated with partial seizures, while infantile spasms are more common in children with TSC. Prophylactic antiepileptic/antiepileptogenic treatment would be an attractive approach to prevent development of seizures in affected children.
There had been two attempts to test prophylactic antiepileptic treatment in children with a neurocutaneous disorder. First, infants with SWS showed a 50% lower rate of epilepsy after prophylactic phenobarbitone administration.22 Second, in a study of 45 infants with TSC, preventive vigabatrin treatment led to an increased rate of seizure freedom (93% vs. 35%), and lower incidence of drug-resistant epilepsy and mental retardation.23 Novel antiepileptic drugs or emerging antiepileptogenic agents could be tested in these patient groups, especially if selected biomarkers could accurately identify those at highest risk for severe, drug-resistant seizures.
In an attempt to identify potential molecular imaging markers of epileptogenesis associated with SWS, we obtained FDG-PET scans in 60 children who were subjects of a prospective, longitudinal neuroimaging study.24 Specifically, we analyzed the clinical correlates of increased cortical glucose metabolism, an interictal PET imaging feature previously observed in some children with SWS.25 Altogether, 9 of the 60 children had increased metabolism on PET (Figure 9.2), while electroencephalography (EEG) during the FDG uptake period did not show seizures or frequent interictal spikes. These children were younger than those who showed decreased glucose metabolism (1.9 vs. 4.5 years, respectively). Also, SWS children scanned before 1 year of age had a higher prevalence of hypermetabolism than older children (33% vs. 9%). Hypermetabolism typically occurred shortly before or after the onset of the first clinical seizure. In longitudinal studies, the hypermetabolic changes invariably switched to hypometabolism. These data suggested that interictal hypermetabolism in SWS is a transient metabolic phenomenon closely associated with imminent or recent onset of clinical seizures. Although these data were preliminary, they raised the intriguing possibility that interictal hypermetabolism in SWS may be an imaging biomarker for epileptogenesis.
Figure 9.2. Glucose metabolic changes in Sturge-Weber syndrome (SWS) associated with epilepsy in an 11-month-old child. The leptomeningeal malformation affected the right hemisphere. (A) Interictal hypermetabolism in the right frontal cortex (solid arrow), already present before the onset of the first clinical seizure. Slightly increased metabolism is also seen in the right parietal region. (B) Follow-up PET scan at 3 years of age showed progression with marked hypometabolism in areas that were hypermetabolic on the initial scan (dotted arrows). By this age, the child had a history of seizures, which were controlled on antiepileptic medication.
The exact mechanisms of the interictal increase of glucose metabolism during the early stage of SWS need to be clarified. A plausible mechanism would be the metabolic effect of excess glutamate released as a result of chronic hypoxia due to the SWS-associated venous malformation. Because of the tight coupling between glucose metabolism and glutamate in human epileptic foci, ischemic injury of the cortex in SWS may lead to glutamate excitotoxicity in conjunction with glucose hypermetabolism. The role of excess glutamate in early SWS-induced seizures and hypermetabolism is also supported by preliminary MR spectroscopic findings demonstrating increased glutamate levels in the affected brain regions.26 Similar increases in glutamate/glutamine levels have been reported in the cerebral cortex of children after traumatic brain injury.27 These data suggest that pharmacologic interventions targeting glutamate may be beneficial to block the detrimental effects of excess glutamate and perhaps halt epileptogenesis in certain forms of human epilepsy.
9.3.2 Glucose Metabolist Changes in Recent-Onset Epilepsy
Studies of brain glucose metabolism in patients with new-onset seizures are scarce. In a study of 40 children who underwent FDG-PET scanning within one year after their third unprovoked seizure, focal hypometabolism was observed only in 20%, with abnormalities confined to the temporal lobe ipsilateral to their presumed epileptic focus.28 This incidence was much lower than hypometabolism reported in patients with chronic “medically-controlled” epilepsy (48% abnormal PET)29 and those with intractable epilepsy where focal hypometabolism is present in the majority of cases. In a subsequent, longitudinal study of the same investigators, focal hypometabolism on the initial PET was associated with poor subsequent seizure control.30 In addition, longer duration of epilepsy before the first PET scan was associated with subsequent development of hypometabolism on follow-up PET. These studies support the notion that early focal metabolic abnormalities, similar to early brain lesions visualized on structural imaging, indicate a risk for uncontrolled epilepsy. In this regard, early focal hypometabolic regions may be considered an imaging marker of subsequent intractability.
9.3.3 Glucose Metabolic Changes in Intractable Epilepsies
The vast majority of human glucose PET studies in epilepsy has been performed in populations with intractable seizures, often in surgical series. These subjects typically have a longstanding history of uncontrolled seizures, whose detrimental effects on brain function and structure may mask metabolic abnormalities specifically linked to epileptogenesis. In the majority of these patients, including those with TLE and extratemporal epilepsies, FDG-PET shows metabolic abnormalities in the form of interictal hypometabolism; the distribution of these metabolic changes largely depends on the type of epilepsy and the location of the epileptic focus.31, 32 Altogether, the majority of patients with medically intractable epilepsy show areas of hypometabolism in the epileptic hemisphere on interictal FDG-PET. Such hypometabolic areas, however, are not specific for epileptogenic regions (as defined by EEG), as they often extend to nonepileptic cortex and also subcortical structures such as the ipsilateral thalamus. Indeed, while the exact pathophysiology of impaired energy metabolism in epileptic brain regions remains unclear, hypometabolism can be present in any brain region showing neuronal loss or chronic synaptic deafferentation.32 A recent study has also demonstrated mitochondrial dysfunction (reduced complex IV functioning) in resected brain tissue that showed PET hypometabolism before surgery in patients with intractable epilepsy and cortical dysplasia.33
Presence of focal hypometabolism is less frequent but not uncommon in nonselective epilepsy cohorts, including patients with medically controlled seizures. In a large, prospective series of 700 patients, Swartz et al.34 found focal or regional FDG-PET abnormalities in 41–63% of cases depending on the lobar localization of the epileptic focus. In the nonsurgical subgroup, this rate varied between 28–37%. Altogether, these data suggested that patients with long-standing, drug-resistant epilepsy have more common (and more severe) brain metabolic abnormalities than those in an early stage of clinical epilepsy and epilepsies that can be controlled by antiepileptic drugs.
A small, longitudinal FDG-PET study, presented by our group, further supported the notion that cortical glucose hypometabolism in the epileptic brain can be progressive if seizures remain uncontrolled. In this study of 15 children with nonlesional epilepsy, the extent of cortical glucose hypometabolism measured in the epileptic hemisphere in 7–44 months intervals expanded in most patients who had persistent or increasing seizure frequency between the PET scans35 (Figure 9.3). In contrast, decreased seizure frequency was accompanied by a decrease in the size of hypometabolic cortex. Similarly, dynamic glucose metabolic changes, associated with seizure frequency between scans, were observed in children with lesional neocortical epilepsy due to SWS.36
Figure 9.3. Progressive expansion of cortical hypometabolism in medically uncontrolled neocortical epilepsy. The initial FDG-PET scan (left image) showed a larger area of hypometabolism (projected on the 3D brain surface reconstructed from MRI) in the left parietal, posterior temporal cortex, and additional smaller hypometabolic areas in the frontal lobe. The child had one seizure per day at the time of the first scan, which increased to 10 per day in the next two years. Repeated PET scan 27 months later showed more extensive hypometabolism in the affected left hemisphere (right image).
Hypometabolism in TLE is often not confined to the temporal lobe, but the exact significance of extratemporal metabolic abnormalities remains to be clarified. In a study of 47 patients with intractable unilateral mesial TLE, extratemporal hypometabolism, particularly contralateral to the epileptic focus, was a marker of poor surgical outcome, independent of other prognostic factors such as MRI, pathology, and EEG findings.37 It is also notable that complete removal of the epileptic focus can reverse some of the hypometabolism observed in remote brain regions, as reported in several series. After temporal lobectomy or selective amygdalo-hippocampectomy, postsurgical PET could demonstrate normalization of glucose metabolism in some remote areas apparently connected to the primary (resected) epileptic focus.38–40
In summary, imaging studies in animal models and human epilepsy demonstrate early metabolic changes during the period of epileptogenesis and subsequent progression of hypometabolism if the seizures cannot be adequately controlled. Although the exact mechanisms of abnormal glucose metabolism in and beyond epileptic foci remain to be clarified, some studies suggest a potential role of increased glutamate and abnormal mitochondrial function. Longitudinal studies clearly suggest that some of the hypometabolic changes are the consequence of repeated seizures and are often multifocal and localized in an epileptic network, and some of them can be reversed by long-term seizure control by medication or surgery. Molecular imaging can provide invaluable data on metabolic changes during epileptogenesis and could also be useful to evaluate effects of antiepileptogenic interventions in the future.
9.4 Molecular Imaging Correlates of Neuroinflammation
Activation of inflammatory pathways is increasingly implicated in the pathophysiology of human epilepsy.8 Finding imaging markers to detect epilepsy-associated neuroinflammation would be important to identify epileptogenic lesions and foci amenable to anti-inflammatory treatment and also to monitor treatment effects in clinical trials. Glucose metabolism is generally decreased in epileptogenic brain regions showing low-level chronic inflammation. On clinical FDG-PET imaging, epileptogenic inflammatory lesions almost invariably display hypometabolism and cannot be reliably differentiated from most other epileptogenic etiologies.41 The rare exception could be severe epilepsies associated with autoimmune encephalitis, where FDG-PET can be useful by showing areas of increased metabolism.42 Still, in most common cases, imaging modalities specifically targeting various aspects of inflammatory processes are needed to capture neuroinflammatory components of epilepsy. Some of the promising imaging modalities already have been tested and proven to be potentially useful in various subgroups of human epilepsy to evaluate neuroinflammation associated with epileptogenesis (Table 9.1). Numerous other molecular imaging probes hold the promise for similar or additional benefit in studying epileptic foci (reviewed recently by Amhaoul et al.9), but most of these await human application. In the next section, we briefly review key results in both animal models of epilepsy and human applications while focusing on two molecular imaging approaches that showed promise in clinical applications: PET imaging of activated microglia and imaging of tryptophan metabolism via the kynurenine pathway.
Table 9.1 PET Radiotracers with Reports of Application in Detection of Neuroinflammation in Epileptic Foci or Lesions
Target/radiotracers | Human disease applications | Limitations |
---|---|---|
TSPO—activated microglia | ||
[11C]-PK11195 | Status epilepticus | Short half-life, high nonspecific binding |
Rasmussen’s encephalitis | ||
Cortical dysplasia | ||
[11C]-PBR28 | Temporal lobe epilepsy | Short half-life, affinity depends on TSPO gene polymorphism |
Neurocysticercosis | ||
[18F]-PBR111 | None (only rat TLE model) | Affinity depends on TSPO gene |
polymorphism | ||
Tryptophan metabolism via the kynurenine pathway | ||
α[11C]-methyl-L-tryptophan | Tuberous sclerosis | Short half-life, limited sensitivity |
(AMT) | Cortical dysplasia | |
Epileptogenic tumors |
TSPO = 18-kDa translocator protein.
9.4.1 Neuroinflammation in Animal Models of Epileptogenesis
PET imaging of activated microglia allows the study of brain inflammation during early epileptogenesis in experimental models. The most commonly used PET radiotracers bind to the translocator protein (TSPO, first described as the peripheral benzodiazepine receptor [PBR]), an 18 kDa protein localized mostly on the outer mitochondrial membrane of activated microglia and also other cells, such as reactive astrocytes. A rat study of the post-kainic-acid-induced SE model for TLE used [18F]-PBR111 PET/CT for this purpose.43 [18F]-PBR111 is a PET radioligand with high specificity for the TSPO.44 Animals with severe SE demonstrated much stronger expression of TSPO in the hippocampus and amygdala in vitro than those with mild symptoms. This TSPO overexpression was corroborated by increases in [18F]-PBR111 volume of distribution in the same brain regions (Figure 9.4). This study provided proof-of-concept data that brain regions critical for seizure generation undergo substantial inflammatory changes during epileptogenesis in this seizure model.
Figure 9.4. PET imaging of brain inflammation during early epileptogenesis in rat. High [18F]-PBR binding indicated activated microglia in the amygdala, thalamus, and hippocampus 7 days after post-kainic-acid-induced status epilepticus (SE).
In a more recent study, PET imaging was used to study the potential significance of abnormal TSPO binding in drug resistance in a chronic animal model of TLE.45 The authors measured brain uptake of [11C]-PK11195 in rats with selection of phenobarbital responders vs. nonresponders. While responders showed uptake values similar to nonepileptic animals, nonresponders showed increased uptake of 26–39% in different brain regions, including hippocampus, putamen, occipital, and parietal cortices. Hippocampal uptake also showed a moderate correlation with seizure frequency. Interestingly, while hippocampi also showed signs of neurodegeneration and expression of activated microglia in both the responder and nonresponder epileptic groups, glucose uptake (measured by PET) did not differ between nonepileptic controls, drug responders, and nonresponders. This study provided evidence for chronic neuroinflammatory changes in the chronic TLE model and raised the possibility that a higher degree of inflammation is associated with drug resistance. However, the observed increases may also be secondary as a result of higher seizure frequency, and it remained unclear if imaging markers of neuroinflammation could predict drug resistance prospectively.
9.4.2 Imaging Activated Microglia in Humans
Initial human PET studies used the PET radioligand [11C]-PK11195 to detect inflammatory changes, in the form of increased binding, in patients with Rasmussen’s encephalitis.46 Increased TSPO binding was detected in the affected hemisphere in two patients. The study demonstrated the feasibility of using TSPO imaging to select potential biopsy site and perhaps assess efficacy of anti-inflammatory treatment. On the other hand, no increased binding was found in the epileptic hippocampus in the same study. In a subsequent study, increased [11C]-PK11195 binding was also reported in the left temporo-occipital cortex of a patient with severe, refractory seizures due to encephalitis of unknown origin.47 In this case the focal PET findings helped in guiding the resection to control seizures.
Despite these promising studies in patients with severe epilepsy associated with encephalitis, molecular imaging studies targeting activated microglia in human epilepsy remained few and far between in the last decade, perhaps because of the relative insensitivity of [11C]-PK11195 to detect low-level brain inflammation as a result of low specific-to-nonspecific binding.48 One case report showed images of increased PK binding in a frontal lobe focus associated with cortical dysplasia,49 with histopathology confirming activated microglia; but this patient also had a history of severe uncontrolled seizures with partial SE. The relatively low sensitivity of [11C]-PK11195 may be overcome by the use of radioligands with better specific binding, such as [11C]-PBR28. Using this radioligand, a preliminary study evaluated TSPO binding in 16 patients with unilateral TLE compared to values of 30 healthy subjects.50 Increased binding was detected in multiple regions ipsilateral to the seizure focus, including the hippocampus, parahippocampal gyrus, amygdala, fusiform gyrus. Interestingly, the asymmetry was more pronounced in patients with hippocampal sclerosis. A limitation of [11C]-PBR28 is that its affinity depends on a single polymorphism in the TSPO gene.
Neurocysticercosis is one of the most common causes of focal epilepsy worldwide. Human neurocysticercosis and related animal models provide a unique opportunity to study mechanisms of epileptogenesis, including epilepsy-related neuroinflammation.51 Calcified granulomas in neurocysticercosis are often associated with perilesional epilepsy and seizures. A PET study with [11C]-PBR28 of nine patients with MRI-proven neurocysticercosis demonstrated increased binding by a mean of 13% in perilesional edema or degenerating cysts.52 This increased binding persisted for 2–9 months in these patients, even after resolution of the edema. The study suggested that anti-inflammatory treatment may be useful to prevent or treat seizures in such patients.
9.4.3 Imaging Tryptophan Uptake in Humans
PET imaging of amino acid uptake is mostly used in clinical brain tumor imaging. Among the most commonly used amino acid PET tracers, O-(2-[18F]fluoroethyl)-L-tyrosine (FET) shows high tumor-specific accumulation (related to upregulated amino acid transport in tumor tissue), while its uptake in inflammatory tissue is negligible.53, 54 [11C]methionine, another frequently used clinical PET tracer for brain tumor imaging, was shown to accumulate in both tumor and inflammatory cells.54 However, its potential use for detecting nontumoral epileptogenic lesions (such as cortical dysplasia) is not well established because of scarce reports with mixed results in such lesions.55–57 In contrast, a wealth of data supports the clinical utility of alpha[11C]methyl-L-tryptophan (AMT) PET for detecting a variety of epileptic foci and lesions in humans; these data are briefly summarized below.
The initial intent of developing a PET radioligand for imaging tryptophan metabolism was to track and quantify serotonin synthesis in the living brain, a valuable goal in neuropsychiatric disorders associated with abnormal serotonergic function. This has been accomplished with the radiosynthesis and subsequent validation of AMT.58, 59 AMT is transported across the blood-brain barrier by specific carriers (most notably the L-type amino acid transporter [LAT]), but it is not incorporated into proteins. Once in the cell, AMT is the substrate of the initial enzymatic steps of serotonin synthesis and is accumulated in serotonergic neurons in the form of alpha-methyl-L-serotonin. Thus, kinetic analysis of AMT uptake allows estimation of serotonin synthesis rate in the living human brain.60
The application of AMT-PET in human epilepsy was initially motivated by studies reporting increased serotonergic immunoreactivity in human epileptic brain tissue associated with cortical dysplasia.61 However, subsequent studies of resected epileptic tissue, showing increased AMT uptake on PET, demonstrated that AMT accumulation in epileptic foci is more related to tryptophan metabolism via the inflammatory kynurenine pathway and may be an imaging marker of epilepsy-associated neuroinflammation.62, 63 Based on studies demonstrating increased levels of epileptogenic and neurotoxic metabolites of the kynurenine pathway (most notably, quinolinic acid, an NMDA receptor agonist), it has been long postulated that activation of this pathway plays a role in epileptogenesis.64, 65 Interest in this pathway has intensified in the last decade following reports showing that activation of this pathway, mostly due to induction of its key enzymes, such as indoleamine 2,3-dioxygenase (IDO) and tryptophan 2,3-dioxygenase (TDO), can lead to tumoral immune resistance.66, 67 Fueled by this interest, a number of potent enzyme inhibitors have been developed and are being tested clinically to block the activity of this pathway and reverse tumoral immune tolerance.68 The results of these efforts may not only benefit tumor treatment but also provide novel therapeutic avenues in human epilepsy associated with inflammation.
The most extensively studied and validated clinical application of AMT-PET was the identification of epileptogenic lesions in patients with TSC. As reported initially69 and confirmed by subsequent studies by multiple groups,70–72 increased focal AMT uptake in the interictal state is a highly specific marker of epileptogenicity in TSC. In the largest study thus far (with 191 TSC patients included), excellent agreement was found between lateralizing ictal scalp EEG and AMT-PET; in 28 of 68 patients (41%) with lateralizing findings, AMT-PET was more localizing.72 In addition, AMT-PET was localizing in 58% of the cases with nonlateralized ictal EEG. “Hot spots” on AMT uptake are not always confined to MRI-defined cortical tubers but can also extend to adjacent dysplastic epileptic cortex.73 Importantly, resection of tubers (or dysplastic lesions) showing increased AMT uptake was associated with seizure-free surgical outcome.73
After the initial success of epileptic lesion localization in TSC, AMT-PET was successfully applied in other epilepsy populations with various etiologies. Most notably, studies in neocortical epilepsy demonstrated the ability of AMT-PET to detect epileptic cortical malformations, such as focal cortical dysplasia.74, 75 A subsequent, detailed comparison to histopathology suggested that, within various histologic types of cortical dysplasia, tryptophan accumulation on PET may be most specific to Type IIB cortical dysplasia.76 Moderate increases in AMT uptake have also been reported in TLE with normal hippocampal volumes,77 but the added clinical value of AMT-PET in presurgical localization of TLE remains unexplored.
While specificity of focal increased AMT uptake for epileptic cortex is very high, its sensitivity is suboptimal and depends on the underlying etiology of epilepsy. The highest sensitivity was observed in dysembryoplastic neuroepithelial tumors (DNTs) and TSC cases, where the epileptogenic lesions can be identified in up to 80% of the cases if the AMT scans are analyzed quantitatively together with other imaging modalities (MRI and FDG-PET).78, 79 The lowest sensitivity was detected in nonlesional neocortical epilepsies, where histopathology could not detect any specific lesion: in those cases, AMT-PET shows increased cortical uptake in less than 50% of the cases.74, 75 A preliminary study with direct comparison of AMT-PET vs. PET imaging of the GABAA receptors (using [11C]-flumazenil, performed in the same patients) also raised the possibility that these two molecular imaging tracers capture different aspects of epileptogenicity at different stages of clinical epilepsy:80 while high AMT uptake was more common in younger patients with shorter epilepsy duration (Figure 9.5), decreased flumazenil uptake was often observed in older children with longer duration of intractable seizures. Altogether, the data thus far suggest that clinical utility of AMT-PET may depend on both underlying etiology and stage of chronic epilepsy. It is also clear that increased AMT uptake provides useful added information in delineation of cortical epileptic foci in important subgroups of patients with intractable epilepsy.
Figure 9.5. Focal increase of tryptophan uptake on alpha[11C]-methyl-L-tryptophan (AMT) PET in a young child with an epileptic focus in the temporal cortex (arrow). [11C]flumazenil PET in the same child showed no focal binding abnormalities.
Recent studies provide emerging evidence that increased AMT uptake in epileptogenic lesions is likely related to chronic inflammatory processes activated in epileptogenic lesions. Indeed, the three most common epileptogenic lesions showing tryptophan accumulation on PET (TSC, DNT, and Type II FCD) all express inflammatory markers such as interleukin-6 or interleukin-1β.81–84 Proinflammatory cytokines, particularly interferon-γ but also IL-1β, can potentiate induction of IDO, thus leading to enhanced metabolism of tryptophan via the kynurenine pathway; this could lead to accumulation of both anti- and proepileptic kynurenine metabolites. To support this mechanism, increased expression of IDO, along with the LAT1 transporter, has been demonstrated in epileptogenic DNTs showing high AMT uptake on PET.79
In order to further establish the link between inflammation, activated kynurenine pathway, increased in vivo tryptophan uptake, and epileptogenicity, we performed an in-depth analysis of imaging, intracranial EEG, and immunohistochemical abnormalities of an inflammatory epileptogenic lesion associated with new-onset refractory SE.85 In this patient, MRI performed after the onset of severe partial seizures showed a tumor-like lesion in the left temporal lobe, displaying T2/FLAIR hyperintensity without contrast enhancement (Figure 9.6). AMT-PET imaging showed high uptake in the lesion area extending behind it to the posterior temporal cortex. The extent of the epileptogenic region was mapped by chronic subdural EEG recording, which showed seizure onset in the left temporal cortex overlapping with the AMT-positive region. Analysis of brain tissue sampled from this region demonstrated reactive astrocytosis, microglial activation and sparse lymphocytic inflammation, along with coexpression of IDO, IL-1β, and the IL-1β receptor (Figure 9.6). Sampling of tissue away from the AMT hot spot showed minimal expression of the same inflammatory markers. Extensive left temporal resection removed the inflammatory lesion along with the bulk of AMT-positive epileptic cortex. Postsurgical follow-up demonstrated long-term seizure freedom and no increased AMT uptake on PET. This study provided proof-of-principle evidence that high AMT uptake on PET can identify epileptogenic areas/lesions with inflammatory changes associated with an upregulated kynurenine pathway. Further studies with stereotactic, image-guided sampling of epileptic foci are required to establish if this close relation between molecular imaging findings and epilepsy-related inflammation is indeed present and common in focal epilepsies of various etiologies.
Figure 9.6. (A) Increased alpha[11C]methyl-L-tryptophan (AMT) uptake (arrows on AMT-PET image and 3D MRI/PET surface) in the left temporal lobe in an adult patient with new-onset severe epilepsy. MRI showed increased FLAIR signal in the midtemporal region (without contrast enhancement; arrow). Intracranial EEG monitoring showed left temporal cortical seizure onset: affected electrodes are overlaid onto 3D PET/MRI surface, where cortex with high AMT uptake is in red. (B, C) Immunohistochemistry from image-guided biopsy specimens showed coexpression of interleukin-1β (IL-1β) and indoleamine 2,3-dioxygenase (IDO) in the AMT+ tissue only.
Acknowledgments
Some of the work presented here is the result of close collaborations with Harry T. Chugani, Diane C. Chugani, Otto Muzik, and other faculty and staff at the PET Center and Translational Imaging Laboratory at Wayne State University and the Children’s Hospital of Michigan. Funding for these studies was provided by US National Institutes of Health, National Institute of Neurological Disorders and Stroke grants NS41922, NS34488, NS38324, and NS64989, and US National Institutes of Health, National Cancer Institute grant CA123451.
References










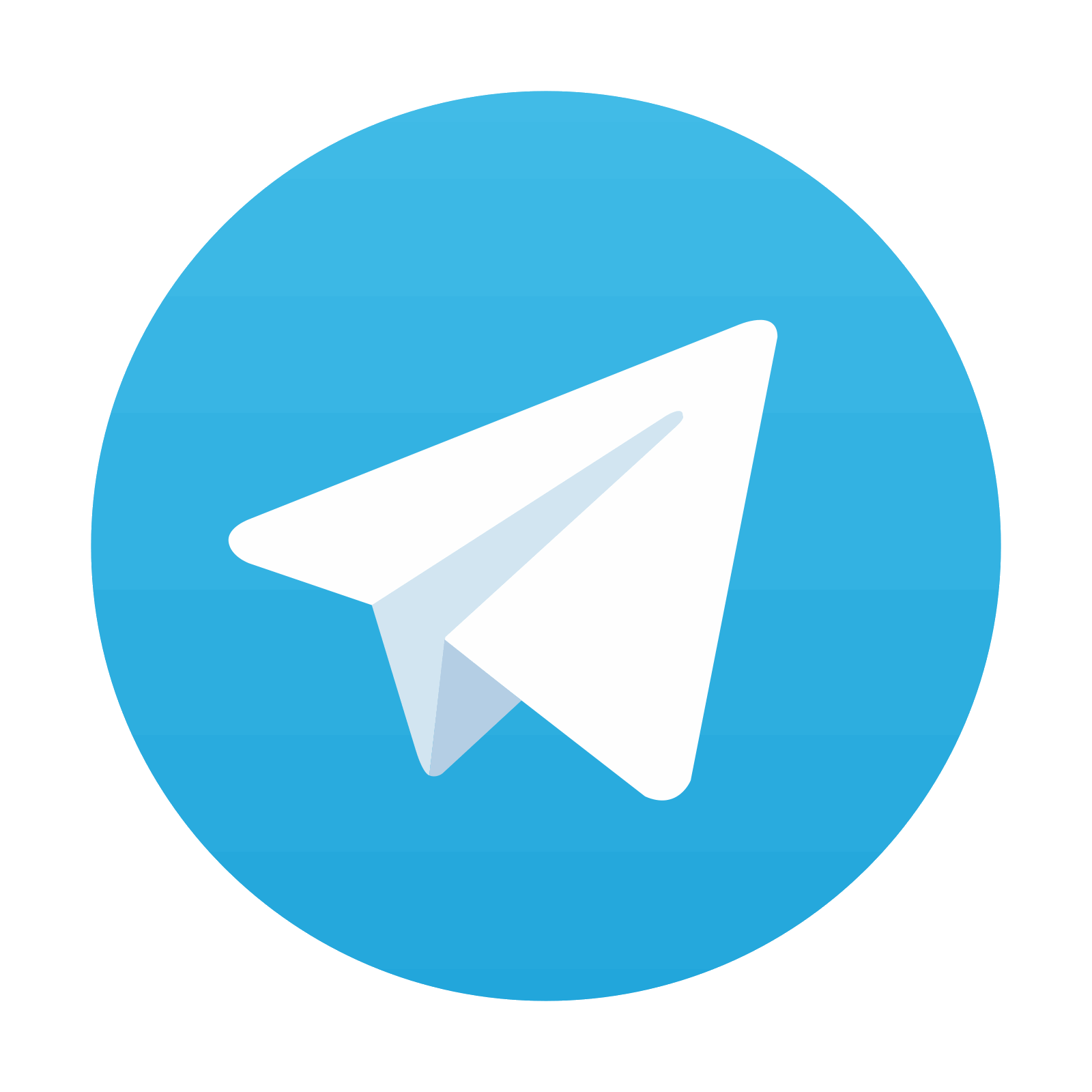
Stay updated, free articles. Join our Telegram channel
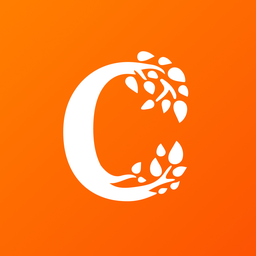
Full access? Get Clinical Tree
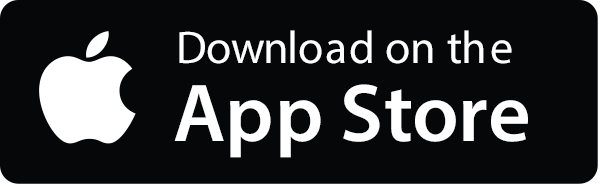
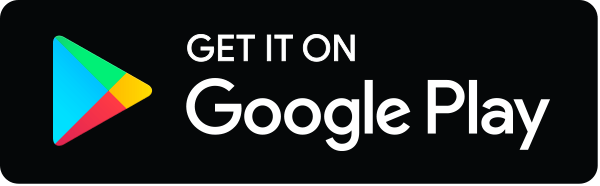
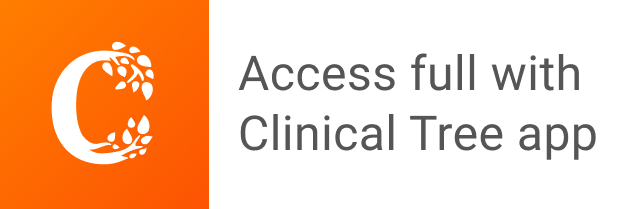