2.1 Introduction
The long-term consequences of a brain trauma and the magnitude of this problem are often not recognized. Epilepsy after traumatic brain injury (TBI) develops only in a subpopulation of patients over several years: after severe injuries, epilepsy develops in 17% of patients.1 There is remarkable variability in terms of severity, brain trauma location, genetic background, and medical history. Therefore, it is currently impossible to predict the outcome of patients after TBI. Imaging biomarker studies of posttraumatic epileptogenesis are very difficult and expensive to perform in patients, as they require long follow-up times in large populations. In practice, identification studies of imaging biomarker candidates in animal models are needed before proceeding to large-scale human studies. This is possible due to dedicated small animal imaging systems and recent development of adequate models for posttraumatic epilepsy. For example, it has recently been shown that only about 30–50% of animals develop epilepsy after experimental fluid-percussion-induced TBI.2, 3
2.2 MRI Applied to Animal Models of Posttraumatic Epilepsy
Magnetic resonance imaging (MRI) is the most versatile in vivo imaging approach available, capable of providing information about anatomical, microstructural, functional, and metabolic changes in the brain. It is based on inherent properties of certain nuclei that are important in biological systems such as 1H, 13C, or 31P. Most often the signal coming from 1H in water molecules is measured. The signal is based on the nuclear magnetic resonance (NMR) phenomenon, and interaction with nuclei takes place in the radio frequency (RF) area of the electromagnetic spectrum. Therefore, MRI does not involve ionizing radiation, has great tissue penetration, and is almost completely noninvasive. Due to abundance of water in tissue, excellent signal-to-noise ratio and high spatial resolution can be obtained. As signal behavior is modulated by complex interaction of water molecules with tissue structures, MRI has an excellent soft tissue contrast. Considering these properties, it is not surprising that MRI has been used in both animal models and clinical settings to assess multiple facets of posttraumatic alterations in the brain. In the following pages, we introduce some conventional and advanced MRI approaches that have been applied to animal models of TBI and posttraumatic epilepsy to identify potential MRI biomarkers for epileptogenesis. Most of these approaches can be translated to human studies with relatively small modifications.
2.2.1 Specific Features of Small Animal MRI
The human brain weighs ~700 times more than the rat brain. As the water content in both human and rat brains is approximately the same, the amount of MRI signal coming from water is ~700 times larger in the human brain. It is evident that more sensitive MRI scanners are needed for rodent MRI studies to compensate for this difference. Animal MRI scanners typically have a higher magnetic field (4.7 T–16 T) than human scanners, which leads to higher initial polarization and, together with small, dedicated RF coils, helps to compensate for smaller signal. As a result, similar or even better anatomical resolution can be achieved in rodents than in humans. The higher magnetic field also brings in some technical challenges. Artifacts coming from different magnetic susceptibilities in the tissue interfaces are more severe and relaxation times are different from those in human MRI. These technical challenges can be addressed with higher magnetic field gradients, strong shims, and pulse-sequence designs, but MRI techniques used in animal studies often require more tailoring by experienced MRI physicists than similar approaches in human studies. Nevertheless, translation of techniques from clinical settings to experimental ones can be done in most cases when the field-strength-dependent factors are taken into account appropriately.
Another specific feature of animal MRI studies is the use of anesthesia needed to immobilize and comfort the animals during the scanning. While this is not commonly an issue for structural imaging, anesthesia can have a profound effect on brain activity, basal blood flow, and neurovascular coupling, hampering functional and hemodynamic imaging studies. Also, there is increasing awareness that anesthesia may influence the outcome of the animals after brain injuries.4
2.2.2 Anatomical MRI and Quantitative Relaxation Time Mapping for Detection of Progressive Tissue Damage
Anatomical imaging is the cornerstone of all MRI protocols. Typical resolution achievable routinely is in order of a few hundred micrometers in plane with 0.5–1.0 mm slice thickness; however, current trend is toward more isotropic voxels that allow better coregistration and group-level analysis. Good contrast between white matter and gray matter and between normal tissue and typical lesion can be obtained with T2 relaxation weighting in high magnetic field used in small animal MRI, while T1 weighting is most often used in clinical MRI. T2 relaxation is an NMR property of nucleus, which for 1H in tissue is predominantly dictated by amount and type of interaction of water molecules with macromolecules in tissue and the presence of strongly paramagnetic or diamagnetic compounds. Therefore, T2-weighted imaging can visualize for example, white matter (high myelin content), edema (high water content), and hemorrhage (high iron content) after TBI. It should be noted that relaxation-based contrast is not very specific and several tissue alterations can lead to apparently similar changes in images. Therefore, interpretation of the results has to be done with caution and may require histological validation.
Volumetric approaches are most commonly exploited in assessment of posttraumatic alterations. Primary lesion area has been found to increase several months after the impact in lateral fluid percussion model in rat.5 Progressive tissue damage can be seen in atrophy of the hippocampus and thalamus and thinning of the cortical structures, which leads to increased volume of ventricles.5, 6 Conventionally, volumetric analysis is based on manual segmentation of the areas of interests, which is time-consuming and susceptible for personal bias. The modern analysis approaches rely on atlas-based coregistration and automated algorithms that allow determination of volumes, thicknesses, and shapes of anatomical structures. Large lesion size and extensive atrophy in many TBI models make automated approaches very challenging, and therefore they have been so far seldom used in experimental studies. However, a recent study showed that hippocampal surface shape could differentiate rats that developed epilepsy after fluid percussion TBI.3
While relaxation time changes after TBI are most often exploited as a source of contrast in T2- or T1-weighted imaging, T2 and T1 are physical constants that can be quantified. Results can be displayed in the form of quantitative relaxation maps. This is especially useful in estimating the progression of the primary lesion. T2 relaxation has two-phasic behavior in most injury models. T2 peaks typically 1–3 days after impact due to vasogenic edema. During the following 1–2 weeks initial edema is reabsorbed and T2 returns toward the baseline value.7 In the chronic phase, after 1 month tissue structures in primary lesion area gradually degrade and eventually lesion in severe cases forms a cavity filled with cerebral spinal fluid (CSF) with very high T2.5, 6
Quantitation of the relaxation times is extremely useful also in biomarker studies. Even though relaxation times are dependent from magnetic field strength, and to a certain extent pulse sequence, with proper harmonization results from different MRI laboratories can be pooled together to reach better statistical power for biomarker analysis. Absolute numerical value of relaxation time can be easily tested, for example, for its predictive value for outcome and included in multiparametric biomarker analysis. This was demonstrated by a recent study showing excellent predictive value of T1ρ relaxation time (rotating frame variant of T1 relaxation) measured in the perilesional cortex after TBI in rats for increased seizure susceptibility (Figure 2.1).6
Figure 2.1. Summary of receiver operating characteristic (ROC) for quantitative relaxation and diffusion parameters assessed at 9 days (A) and 23 days post-injury (B), which predicted increased seizure susceptibility at 12 months post-TBI (traumatic brain injury). A diagonal line represents 50% probability for correct prognosis. T2-weighted image (C), T1ρ map (D), T2 map (E), and ADC map from a representative animal 23 days post-TBI.
2.2.3 Detection of Hemorrhage and Calcification by MRI Sensitive to Magnetic Susceptibility
Hemorrhage is one of the key features associated with TBI also in animal models. Detection of the hemorrhage is based on local magnetic field distortion caused by paramagnetic iron from blood and its degradation products, which have much higher magnetic susceptibility than surrounding tissue. As small animal MRI is generally performed in a high magnetic field which amplifies the effect, MRI has excellent sensitivity to detect hemorrhage in animal models. Large hemorrhages are typically visible already in conventional T2-weighted anatomical images as dark signal void areas. For detection of microhemorrhages gradient echo imaging with T2* weighting with better sensitivity is generally used (Figures 2.2A and 2.2C). The multiecho gradient-echo approach allows quantification of T2* relaxation times and mapping of the magnetic field variation caused by iron. More advanced field-mapping-type approaches (frequency maps, phase maps) allow distinction of paramagnetic substances from diamagnetic ones. For example, diamagnetic calcifications are a common finding in the thalamus after lateral fluid percussion injury, and are often found in the vicinity of hemorrhages. In T2(*)-weighted images, both calcifications and blood appear black, while in field or phase maps opposite dipolar pattern coming from diamagnetic versus paramagnetic compounds can be detected, as recently shown in the fluid percussion TBI model by Lehto et al.9 Susceptibility-weighted MRI (SWI) combines phase image with magnitude image and can help to visualize hemorrhages and has high translational value as it is widely used in clinical studies.10 However, quantification is challenging as the contrast presents a mixture of phase and magnitude contrast. The most advanced technique for detection of susceptibility related changes in brain is quantitative susceptibility mapping (QSM). By solving the magnetic field to source the inverse problem, it is possible to produce maps of magnetic susceptibility of the tissue. QSM allows sensitive visualization and more quantitative assessment of the calcification and hemorrhage (Figures 2.2B and 2.2D). Reconstruction of QSM maps is a multistep pipeline involving background field removal and ill posed field to source inversion with no gold standard approach currently available, especially for animal work. The hemorrhages can also be problematic as incomplete inversion can lead to streaking artifacts spreading signal from the site of hemorrhage to surrounding tissue. Despite these challenges, QSM provides a promising yet rapidly evolving tool for assessment of posttraumatic changes in the rodent brain.
Figure 2.2. Representative T2* (A, C) and QSM maps (B, D) from a sham-operated rat and a rat six months after severe lateral fluid-percussion-induced TBI. White arrowheads point at alterations in susceptibility or relaxation times due to microbleeds, and the white arrow indicates the calcification. Note that in T2* maps both iron and calcium deposits decrease the relaxation time. In QSM maps, areas with high iron content show higher susceptibility values (paramagnetic) and areas with high calcium content have lower susceptibility values (diamagnetic).
2.2.4 Diffusion MRI—Cytotoxic Edema, White Matter Damage, and Beyond
MRI can be sensitized to magnitude and direction of water diffusion by adding a pair of strong magnetic field gradients with opposite polarities into the imaging pulse sequence. The signal coming from water molecules that stay stationary is not changed by bipolar field gradients, while net displacement during the time between gradients leads to signal attenuation. This allows quantification of apparent diffusion coefficient (ADC) of water in tissue. The diffusion coefficient is called “apparent” as diffusion is restricted by surrounding cell and tissue structures. Indeed, this is what makes diffusion a powerful in vivo imaging contrast, as it relays information about water distribution and tissue microstructure rather than just absolute water diffusion constant in given temperature.
The most simple diffusion measurement determines only orientation insensitive ADC in tissue. ADC drops 20–40% within minutes to hours after impact due to cytotoxic edema, when most conventional MRI techniques cannot yet detect any changes in the brain.5, 7 Cytotoxic edema is a result of energy failure in tissue leading to influx of ions followed by water into intracellular space, without net increase of water content in tissue. Diffusion stays decreased from a few hours to several days depending on the animal model and the brain area. Normalization (often called pseudo-normalization as it does not indicate that tissue has recovered) of diffusion is typically followed by increased diffusion weeks—months after the impact when degradation of tissue takes place.5 This characteristic time course, especially when combined with time course of relaxation changes and relaxation measurements, allows monitoring of progression of lesion and severity of the damage. ADC has potential also to serve as biomarker for outcome after TBI.11, 12 It has been shown that ADC measured in hippocampus as early as 3 hours after fluid percussion injury in rat correlated with increased seizure susceptibility in chronic phase 1 year later.12
Nowadays, diffusion is typically measured using at least six different orthogonal orientations of diffusion sensitizing gradient pairs, which allows determination of diffusion tensor and forms the basis for diffusion tensor imaging (DTI). From diffusion tensor, several parameters allowing characterization of TBI-induced tissue changes can be calculated. Most common scalar parameters are fractional anisotropy (FA, which indicates how much diffusion deviates from isotropic diffusion), and axial and radial diffusivity (DII, D⊥, the average amount of diffusion in the main diffusion direction and perpendicular to that, respectively). Decreased FA is used as a nonspecific marker related to the degradation of oriented gray and white matter structures, while DII and D⊥ are most often used to characterize white matter changes in TBI models. Changes in DII are suggested to be more specifically associated with axonal damage and an increase in D⊥ with demyelination.13 There is growing awareness that this general interpretation is probably oversimplified, however, and multiple factors may affect all scalar DTI metrics after TBI. In a recent study, widespread DTI changes in chronic phase after fluid percussion DTI were detected both in gray matter areas and major white matter tracks outside the primary lesion areas colocalizing with neuronal loss, decrease in myelinated fiber density, and iron accumulation.14 Very high resolution ex vivo DTI has been used to detected changes in hippocampal network plasticity after TBI.15 The most robust changes in the CA3 were increased FA and DII and a change in the orientation of the water diffusion, and in the dentate gyrus a decrease in FA and DII. The alterations in DTI parameters were associated with mossy fiber and perforant pathway axonal reorganization. While this study was performed ex vivo, similar changes were recently detected in longitudinal study after status epilepticus,16 showing feasibility to detect damage induced axonal reorganization in vivo (Figures 2.3A and 2.3B).
Figure 2.3. Representative directionally encoded color (DEC) fractional anisotropy maps in vivo (A) and ex vivo (B) diffusion tensor imaging (DTI), and a track-density imaging map (TDI) (C) from the same rat five months after severe fluid-percussion-induced TBI. The white arrowheads indicate increase in FA and changes in water diffusion orientation in CA3 of the hippocampus. The white arrow points to a decrease in FA and track density in the perilesional cortex in the ipsilateral side as compared to the contralateral side. Color coding for directions: green: dorsal-ventral, red: lateral-medial, and blue: rostral-caudal.
Changes in the orientation of the structures measured by DTI can be used to pinpoint post-TBI structural changes,16, 17 but most often orientation information is used for tractography. Modern approaches go beyond classical DTI approach, and use more complex data acquisition and reconstruction strategies allowing separation of crossing fibers. While tractography provides visualization of white matter tracts, some of the related approaches provide whole-brain tractography using super-resolution techniques, such as track-density imaging (TDI) (Figure 2.3C) and quantitative metrics that allow the characterization of microstructural damage generated by TBI. In a recent work, Wright et al. utilized apparent fiber density (AFD) and track-weighted imaging (TWI) metrics such as track density, average path length, and curvature to detect changes in white matter 12 weeks after fluid percussion TBI in rats.18 They showed that AFD and TWI metrics were more sensitive than conventional DTI metrics to posttraumatic changes and could find changes also in corticospinal tract that appeared normal in DTI.
2.2.5 Functional MRI—Detection of Functional Defects and Network Reorganization
Functional MRI (fMRI) detects brain activity indirectly through hemodynamic changes that are tightly coupled to neuronal activity. In the close vicinity of activated brain area, cerebral blood flow (CBF) and volume (CBV) are increased in a way that overcompensates elevated oxygen consumption and leads to an increased oxyhemoglobin to deoxyhemoglobin ratio in the area. As oxyhemoglobin is diamagnetic and deoxyhemoglobin paramagnetic, a shift in the balance can be detected through signal changes in T2- or T2*-weighted images. This forms the basis to so-called blood-oxygenation-level-dependent (BOLD) contrast, which is most often used in both human and animal fMRI. In animal fMRI, CBV imaging exploiting intravascular contrast agents is also commonly used due to its greater contrast-to-noise ratio than BOLD. Nevertheless, CBV fMRI relies on the same neurovascular coupling phenomenon as BOLD imaging.
In classical fMRI, rapid imaging pulse sequences with temporal resolution of 1–2 seconds are used to continuously acquire MRI signal during repeated stimulus and resting periods. Measured MRI signal time courses are statistically compared with expected signal time course obtained by convoluting stimulation paradigm with hemodynamic response function. The fact that fMRI is not a direct measure of neuronal activity, but detects activation through neurovascular coupling, should be taken into account when interpreting fMRI results from TBI models. fMRI changes may not always present changes in brain activity, as TBI-induced changes in basal blood flow, vasculature, and complex cascade behind neurovascular coupling may contribute to detected changes or mask the altered brain activity. The situation is further complicated by possible influence of anesthesia on fMRI response in animal models. In spite of these complications, a recent study showed impaired BOLD fMRI response to fore paw stimulation, in the primary somatosensory area in the rat cortex that appeared intact in structural MRI, 2 days after fluid percussion TBI.19 Interestingly, functional response partially recovered two weeks later. Simultaneous local field potential and fMRI measurement were able to confirm that changes in BOLD fMRI responses were indeed associated with alterations in electrical activity in brain rather than changes in hemodynamics.
The fMRI signal measured without any external stimulation also has time-dependent signal changes arising from multiple different factors including rhythmic changes in underlying brain activity. This so-called resting state fMRI (rs-fMRI) signal can be used to determine functional connectivity between brain regions. The approach is based on the idea that if two brain areas have synchronous changes in resting state brain activity, i.e., their fMRI time series are correlated, the brain areas are functionally connected. The rs-fMRI signal has been extensively used in human TBI studies, and it is increasingly also used in TBI animal models, as it allows large-scale network-level assessment of posttraumatic alterations in brain. This is a highly interesting approach as many long-term outcomes of TBI, including epilepsy, are increasingly recognized to be network-level disorders. In a recent study, rats with increased seizure susceptibility in pentylenetetrazol test, in chronic phase after fluid percussion injury, were studied using rs-fMRI.20 The group statistics revealed decreased connectivity between the ipsilateral and contralateral parietal cortex and between the parietal cortex and hippocampus on the side of injury as compared to sham-operated animals. Injured animals also had abnormal negative connectivity between the ipsilateral and contralateral parietal cortex and other regions. Novel rs-fMRI analyses, such as graph theory analysis, can also quantify topological features of functional networks. Recently, this approach was applied to controlled cortical impact rat TBI model in days 7–28 postinjury.21 Exploratory global network analysis showed changes in network parameters indicative of possible acutely increased random connectivity and temporary reductions in modularity that were matched by local increases in connectedness and increased efficiency among more weakly connected regions. The global network parameters of shortest path length, clustering coefficient, and modularity that were most affected by trauma also scaled with the severity of injury.
As demonstrated also by the examples above, resting state analysis almost always relies on group-level analysis, in order to compensate for inherently weak contrast-to-noise ratio of the approach. Group-level analysis has produced highly interesting and relevant results, shedding light on the network-level mechanism in posttraumatic cascade. However, a major challenge for rs-fMRI is to be able to detect reliably and reproducibly functional connectivity changes in individual subjects, which is a basic requirement for rs-fMRI to be used in the search for a biomarker for posttraumatic alterations.
2.2.6 Contrast Agents and Arterial Spin Labeling—Measuring Blood Flow and Blood-Brain Barrier Leakage
Gadolinum (Gd) chelate T1-contrast agents are widely used to measure hemodynamic parameters such as relative CBF, CBV, and mean transit time (MTT) in both experimental and clinical settings. Gd contrast agents are typically used in the dynamic contrast enhanced (DCE, also called bolus tracking) approach. In this approach, signal intensity changes during first pass bolus of contrast agent are measured using rapid imaging with temporal resolution of 0.5–1 seconds and relative CBV, CBF, and MTT are calculated from signal time courses presenting. In addition, superparamagnetic iron oxide particles are often used in small animal MRI, as they provide very strong T2 and T2* contrast. Due to the large size of these contrast agents, they stay in the intravascular pool for an extended period of time, which makes possible high-resolution mapping of relative CBV. This type of CBV imaging was recently used to characterize temporal profile of CBV in the perilesional area, hippocampus, and primary lesion after weight drop induced TBI in the acute-subacute phase.22 While the first response to injury was a decrease of CBV in acute phase all brain areas, the recovery profile varied in subacute phase including also overshoot of CBV in the hippocampus at 2 weeks. The CBV changes in the subacute phase were associated with motor recovery emphasizing the value of hemodynamic changes as a potential biomarker of functional outcome after TBI.
CBF can also be measured without an external contrast agent using arterial spin labeling (ASL). As this approach provides quantitative CBF values in absolute units and can be used in both animals and humans, it has great potential in the search for a biomarker. The technique is based on noninvasive labeling of inflowing blood with RF pulses, typically in the level of the neck. The small difference in signal intensity between images with labeled and images with nonlabeled inflowing blood is used to calculate CBF maps. Due to small differences in the signal intensity between labeled and nonlabeled images, ASL has inherently lower contrast-to-noise ratio and thus requires a longer acquisition time than the DCE approach with contrast agent. Nevertheless, ASL has been used to characterize longitudinal changes in CBF in the acute-subacute23 and chronic phases24 in posttraumatic epilepsy model.
Impaired integrity of blood-brain barrier (BBB) is an important hallmark of TBI, and it has recently been described as one of the potential mechanisms causing posttraumatic epilepsy.25 BBB integrity can be measured using MRI contrast agents both in animals and in humans, therefore potentially providing a promising translatable imaging biomarker for epileptogenesis. In the conventional approach, T1-weighted images are measured before and after gadolinium-bolus injection. As an intact BBB keeps contrast agent in the vasculature, leakage of Gd to the extraluminal space can be used as a marker for damaged BBB. Using this approach, Frey et al. showed that BBB damage in injured cortex at 72 hours after fluid percussion injury was significantly correlated with EEG-based measures of seizure susceptibility to chemo convulsant challenge 3 months later.11 A recent improvement to the technique that incorporates step-down infusion of gadolinium instead of bolus injection and quantitative relaxation mapping can detect markedly more subtle BBB damage.26
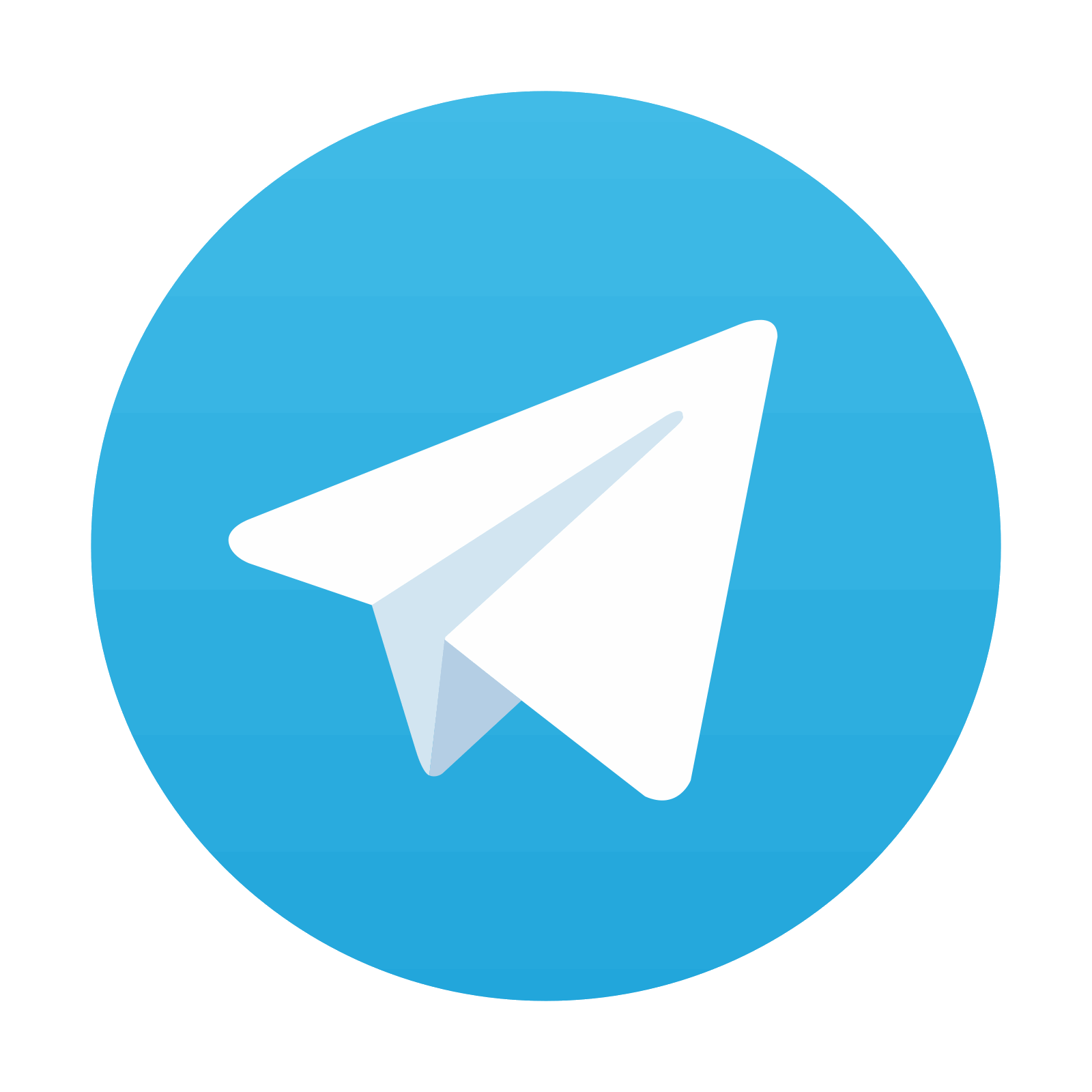
Stay updated, free articles. Join our Telegram channel
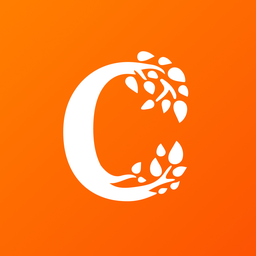
Full access? Get Clinical Tree
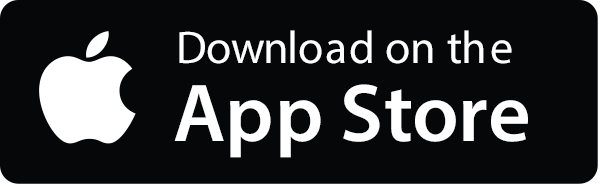
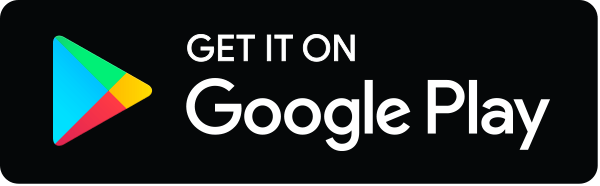
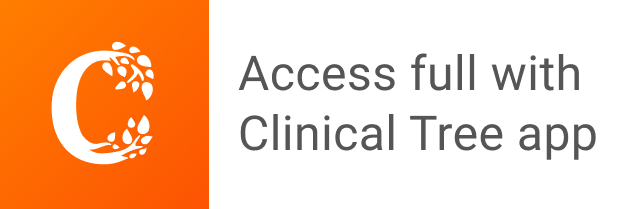