Imaging, Electrophysiologic, and Laboratory Techniques for Neurologic Diagnosis: Introduction
The analysis and interpretation of data elicited by a careful history and examination may prove to be adequate for diagnosis. Special laboratory examinations then do no more than corroborate the clinical impression. However, it happens more often that the nature of the disease is not discerned by “case study” alone; the diagnostic possibilities may be reduced to two or three, but the correct one is uncertain. Under these circumstances, one resorts to ancillary examinations. The aim of the neurologist is to arrive at a final diagnosis by artful analysis of the clinical data aided by the least number of laboratory procedures.
Only a few decades ago, the only laboratory tests available to the neurologist were examination of a sample of cerebrospinal fluid, radiography of the skull and spinal column, contrast myelography, pneumoencephalography, and electroencephalography. The physician’s armamentarium has been expanded to include a multitude of neuroimaging modalities, biochemical, and genetic methods. Some of these new methods give the impression of such accuracy that there is a temptation to substitute them for a careful, detailed history and physical examination. Reflecting the limitations of laboratory diagnosis, in a carefully examined series of 86 consecutively hospitalized neurologic patients reported by Chimowitz and colleagues, laboratory findings (including MRI) clarified the clinical diagnosis in 40 patients but failed to do so in the remaining 46. Moreover, it is common in practice for ancillary testing to reveal abnormalities that are of no significance to the problem at hand. Consequently, the physician should always judge the relevance and significance of laboratory data only in the context of clinical findings. Hence the neurologist must be familiar with all laboratory procedures relevant to neurologic disease, their reliability, and their hazards.
What follows is a description of laboratory procedures that have application to a diversity of neurologic diseases. Procedures that are pertinent to a particular symptom complex or category of disease—e.g., audiography to study deafness; electronystagmography (ENG) in cases of vertigo; electromyography (EMG) and nerve conduction studies, as well as nerve and muscle biopsy, where there is neuromuscular disease—are presented in the chapters devoted to these disorders.
Lumbar Puncture and Examination of Cerebrospinal Fluid
The information yielded by examination of the cerebrospinal fluid (CSF) is crucial in the diagnosis of certain neurologic diseases, particularly infectious and inflammatory conditions, subarachnoid hemorrhage, and processes that alter intracranial pressure. Combinations of findings, or formulas, in the CSF generally denote particular classes of disease; these are summarized in Table 2–1.
CONDITION | CELLS | PROTEIN | GLUCOSE | OTHER FEATURES |
---|---|---|---|---|
Bacterial infection | WBC <50/mm3, often greatly increased | 100–250 mg% | 20–50 mg%; usually lower than half of blood glucose level | Gram stain shows organisms; pressure increased |
Viral, fungal, spirochetal infection | WBC 10–100/mm3 | 50–200 mg% | Normal or slightly reduced | Special culture techniques required; pressure normal or slightly increased |
Tuberculous infection | WBC >25/mm3 | 100–1,000 mg% | >50, often markedly reduced | Special culture techniques and PCR may be needed to detect organisms |
Subarachnoid hemorrhage | RBC >500/mm3; slight increase in WBC | 60–150 mg% | Normal; slightly reduced later | Must be distinguished from traumatic lumbar puncture by presence of xanthochromia of spun sample; greatly increased pressure |
Cerebral hemorrhage, trauma | RBC 50–200/mm3; higher if ventricular rupture of blood | 50–150 mg% | Normal | Pressure may be elevated |
Ischemic stroke | Normal or few WBC | Normal | Normal | Normal pressure unless brain swelling |
Multiple sclerosis | Normal or few WBC | Normal or slightly increased | Normal | Increased IgG fraction and oligoclonal bands |
Meningeal cancer | WBC 10–100/mm3 | Usually elevated | Normal or depressed | Neoplastic cells in CSF; elevation of certain protein markers (e.g., β2-microglobulin) |
To obtain pressure measurements and procure a sample of the CSF for cellular, cytologic, chemical, and bacteriologic examination.
To aid in therapy by the administration of spinal anesthetics and occasionally, antibiotics or antitumor agents, or by reduction of CSF pressure.
To inject a radiopaque substance, as in myelography, or a radioactive agent, as in radionuclide cisternography.
Lumbar puncture (LP) carries some risks if the CSF pressure is very high (evidenced mainly by headache and papilledema), for it increases the possibility of a fatal cerebellar or transtentorial herniation. The risk is considerable when papilledema is the result of an intracranial mass, but it is much lower in patients with subarachnoid hemorrhage, in hydrocephalus with communication between all the ventricles, or with pseudotumor cerebri, conditions in which repeated LPs may at times be employed as a therapeutic measure. Asymmetric lesions, particularly those near the tentorium or foramen magnum carry a greater risk of herniation precipitated by lumbar puncture. In patients with purulent meningitis, there is also a small risk of herniation, but this is far outweighed by the need for a definitive diagnosis and the institution of appropriate treatment at the earliest moment. With this last exception, LP should generally be preceded by CT or MRI whenever an elevation of intracranial pressure is suspected. If radiologic procedures disclose a mass lesion that is causing displacement of brain tissue toward the tentorial opening or the foramen magnum (the presence of a mass alone is of less concern) and if it is considered essential to have the information yielded by CSF examination, the LP may be performed—with certain precautions. If the pressure proves to be very high—over 400 mm H2O—one should obtain the smallest necessary sample of fluid and then, according to the suspected disease and patient’s condition, administer mannitol or another hyperosmolar agent and ideally, to observe a fall in pressure on the manometer. Dexamethasone or an equivalent corticosteroid may generally also be given in an initial intravenous dose of 10 mg, followed by doses of 4 to 6 mg every 6 h in order to produce a sustained reduction in intracranial pressure. Corticosteroids are particularly useful in situations in which the increased intracranial pressure is caused by vasogenic cerebral edema (e.g., tumor-associated edema).
Cisternal (foramen magnum) puncture and lateral cervical subarachnoid puncture, although safe in the hands of an expert, are too hazardous to entrust to those without experience and do not circumvent the problem of increased intracranial pressure. LP is preferred except in obvious instances of spinal block requiring a sample of cisternal fluid or for myelography above the lesion.
Experience teaches the importance of meticulous technique and proper positioning of the patient. LP should be done under locally sterile conditions. Xylocaine is injected in and beneath the skin, which should render the procedure almost painless. Warming of the analgesic by rolling the vial between the palms seems to diminish the burning sensation that accompanies cutaneous infiltration. The patient is positioned on his side, preferably on the left side for right-handed physicians, with hips and knees flexed, and the head as close to the knees as comfort permits. The patient’s hips should be vertical, the back aligned near the edge of the bed, and a pillow placed under the ear. The puncture is usually easiest to perform at the L3-L4 interspace, which corresponds in many individuals to the axial plane of the iliac crests, or at the interspace above or below. In infants and young children, in whom the spinal cord may extend to the level of the L3-L4 interspace, lower levels should be used. Experienced anesthesiologists have suggested that the smallest possible needle be used and that the bevel be oriented in the longitudinal plane of the dural fibers (see below regarding atraumatic needles). It is usually possible to appreciate a palpable “give” as the needle approaches the dura, followed by a subtle “pop” on puncturing the arachnoid membrane. At this point, the trocar should be removed slowly from the needle to avoid sucking a nerve rootlet into the lumen and causing radicular pain. Sciatic pain during the insertion of the needle indicates that it is placed too far laterally. If the flow of CSF slows, the patient’s head can be elevated slowly. Occasionally, one resorts to gentle aspiration with a small-bore syringe to overcome the resistance of proteinaceous and viscous CSF. Failure to enter the lumbar subarachnoid space after two or three trials usually can be overcome by performing the puncture with the patient in the sitting position and then helping him to lie on one side for pressure measurements and fluid removal. The “dry tap” is more often the result of an improperly placed needle than of obliteration of the subarachnoid space by a compressive lesion of the cauda equina or by adhesive arachnoiditis. In an obese patient, in whom palpable spinal landmarks cannot be appreciated, or after several unsuccessful attempts in any patient, fluoroscopy can be employed to position the needle.
LP has few serious complications. The most common is headache, estimated to occur in one-third of patients, but in severe form in far fewer. Prolonged or severe post-lumbar puncture headache is usually seen in patients with a history of migraine. The pain is presumably the result of a reduction of CSF pressure from leakage of fluid at the puncture site and tugging on cerebral and dural vessels as the patient assumes the erect posture. Although neither recumbency nor oral fluid administration after LP has been shown to prevent headache, they are often implemented nonetheless. Strupp and colleagues have found that the use of an atraumatic needle almost halved the incidence of headache. Curiously, headaches are twice as frequent after diagnostic LP as they are after spinal anesthesia. Patients who are prone to frequent headaches before LP reportedly have higher rates of headache afterwards, which accords with our experience. Severe headache can be associated with vomiting and mild neck stiffness. Unilateral or bilateral sixth nerve or other cranial nerve palsies occur rarely after lumbar puncture, even at times without headache and rare cases of hearing loss or facial palsy have been reported. The syndrome of low CSF pressure, its treatment by “blood patch,” and other complications of lumbar puncture are considered further in Chap. 30.
Bleeding into the spinal meningeal or epidural spaces after lumbar puncture can occur in patients who are taking anticoagulants (generally with an international normalized ratio [INR] >1.4), have low platelet counts (<50,000/mm3), or impaired platelet function (alcoholism, uremia). Treatment is by reversal of the coagulopathy and, in some cases, surgical evacuation of the clot. Purulent meningitis and disc space infections rarely complicate LP as the result of imperfect sterile technique, and the introduction of particulate matter (e.g., talc) or irritant carriers of injected drugs can produce a sterile meningitis.
Once the subarachnoid space has been entered, the pressure and fluctuations with respiration of the CSF are determined, (see below) and samples of fluid are obtained. The gross appearance of the fluid is noted, after which the CSF, in separate tubes, can be examined for (1) number and type of cells and presence of microorganisms by direct observation; (2) protein and glucose content; (3) tumor cells (cytology); (4) presence of oligoclonal bands or content of gamma globulin and other protein fractions, and serologic tests; (5) pigments, lactate, LDH, and substances elaborated by some tumors (e.g., β2 microglobulin); and (6) bacteria and fungi (by culture), cryptococcal antigen, mycobacteria, the DNA of herpesvirus, cytomegalovirus and other organisms (by polymerase chain reaction), markers or certain infections (e.g., 14-3-3 protein), and viral isolation.
With the patient in the lateral decubitus position, the CSF pressure is measured by a manometer attached to the needle in the subarachnoid space. In the normal adult, the opening pressure varies from 100 to 180 mm H2O, or 8 to 14 mm Hg. In children, the pressure is in the range of 30 to 60 mm H2O. A pressure above 200 mm H2O with the patient relaxed and legs straightened reflects increased intracranial pressure. In an adult, a pressure of 50 mm H2O or below indicates intracranial hypotension, generally caused by leakage of spinal fluid or systemic dehydration. When measured with the needle in the lumbar sac and the patient in a sitting position, the fluid in the manometer rises to the level of the cisterna magna (pressure is approximately double that obtained in the recumbent position). It fails to reach the level of the ventricles because the latter are in a closed system under slight negative pressure, whereas the fluid in the manometer is influenced by atmospheric pressure. Normally, with the needle properly placed in the subarachnoid space, the fluid in the manometer oscillates through a few millimeters in response to the pulse and respiration and rises promptly with coughing, straining, and with jugular vein or abdominal compression. An apparent low pressure can also be the result of a needle aperture that is not fully within the subarachnoid space; this is evidenced by the lack of expected fluctuations in pressure with these maneuvers.
The presence of a spinal subarachnoid block was in the past confirmed by jugular venous compression (Queckenstedt test, which tests for a rapid rise in CSF pressure within a few seconds after application of the pressure on the vein). The maneuver risks worsening of a spinal block or of raised intracranial pressure and is of historical interest.
Normally, the CSF is clear and colorless. Minor degrees of color change are best detected by comparing test tubes of CSF and water against a white background (by daylight rather than by fluorescent illumination) or by looking down into the tubes from above. (A microhematocrit tube is too narrow for this purpose.) The presence of red blood cells imparts a hazy or ground-glass appearance; at least 200 red blood cells (RBCs) per cubic millimeter (mm3) must be present to detect this change. The presence of 1,000 to 6,000 RBCs per cubic millimeter imparts a hazy pink to red color, depending on the amount of blood; centrifugation of the fluid or allowing it to stand causes sedimentation of the RBCs. Several hundred or more white blood cells (WBCs) in the fluid (pleocytosis) may cause a slight opaque haziness.
A traumatic tap, in which blood from the epidural venous plexus has been introduced into the spinal fluid, may seriously confuse the diagnosis if it is incorrectly interpreted to indicate a preexistent subarachnoid hemorrhage. To distinguish between these two types of “bloody taps,” two or three serial samples of fluid should be taken at the time of the LP. With a traumatic tap, there is usually a decreasing number of RBCs in the subsequent tubes. Also with a traumatic tap, the CSF pressure is usually normal, and if a large amount of blood is mixed with the fluid, it will clot or form fibrinous webs. These are not seen with preexistent hemorrhage because the blood has been greatly diluted with CSF and defibrinated by enzymes in the CSF. In subarachnoid hemorrhage, the RBCs begin to hemolyze within a few hours, imparting a pink-red discoloration (erythrochromia) to the supernatant fluid; if the spinal fluid is sampled more than a day following the hemorrhage, the fluid will have become yellow-brown (xanthochromia). Prompt centrifugation of bloody fluid from a traumatic tap will yield a colorless supernatant; only with large amounts of venous blood (RBC more than 100,000/mm3) will the supernatant fluid be faintly xanthochromic due to contamination with serum bilirubin and lipochromes.
The fluid from a traumatic tap should contain one or two WBCs per 1,000 RBCs assuming that the hematocrit is normal, but in reality this ratio varies widely. With subarachnoid hemorrhage, the proportion of WBCs rises as RBCs hemolyze, sometimes reaching a level of several hundred per cubic millimeter; but the vagaries of this reaction are such that it, too, cannot be relied upon to distinguish traumatic from preexistent bleeding. The same can be said for crenation of RBCs, which occurs in both types of bleeding.
Why red corpuscles undergo rapid hemolysis in the CSF is not clear. It is surely not because of osmotic differences, as the osmolarity of plasma and CSF is essentially the same. Fishman suggested that the low protein content of CSF disequilibrates the red cell membrane in some way.
The pigments that discolor the CSF following subarachnoid hemorrhage are oxyhemoglobin, bilirubin, and methemoglobin. In pure form, these pigments are colored red (orange to orange-yellow with dilution), canary yellow, and brown, respectively. Oxyhemoglobin appears within several hours of hemorrhage, becomes maximal in approximately 36 h, and diminishes over a 7- to 9-day period. Bilirubin begins to appear in 2 to 3 days and increases in amount as the oxyhemoglobin decreases. Methemoglobin appears when blood is loculated or encysted and isolated from the flow of CSF. Spectrophotometric techniques can be used to distinguish the various hemoglobin breakdown products and thus determine the approximate time of bleeding.
Not all xanthochromia of the CSF is caused by hemolysis of RBCs. With severe jaundice, both conjugated and unconjugated bilirubin diffuse into the CSF. The quantity of bilirubin in the CSF ranges from one-tenth to one-hundredth that in the serum. Elevation of CSF protein from any cause results in a faint opacity and xanthochromia, more or less in proportion to the albumin-bound fraction of bilirubin. Only at protein levels greater than 150 mg/100 mL does the coloration become visible to the naked eye. Hypercarotenemia and hemoglobinemia (through hemoglobin breakdown products, particularly oxyhemoglobin) also impart a yellow tint to the CSF, as do blood clots in the subdural or epidural space of the cranium or spinal column. Myoglobin does not enter the CSF because a low renal threshold for this pigment permits rapid clearing from the blood.
During the first month of life, the CSF may contain a small number of mononuclear cells. Beyond this period, the CSF is normally nearly acellular (i.e., fewer than 5 lymphocytes or other mononuclear cells per cubic millimeter). An elevation of WBCs in the CSF always signifies a reactive process to bacteria or other infectious agents, blood, chemical substances, an immunologic inflammation, a neoplasm, or vasculitis. The WBCs can be counted in an ordinary counting chamber, but their identification requires centrifugation of the fluid and a Wright stain of the sediment or the use of a Millipore filter, cell fixation, and staining. One can then recognize and count differentially neutrophilic and eosinophilic leukocytes (the latter being prominent in Hodgkin disease, some parasitic infections, neurosyphilis, and cholesterol emboli), lymphocytes, plasma cells, mononuclear cells, arachnoid lining cells, macrophages, and tumor cells. Bacteria, fungi, and fragments of echinococci and cysticerci can also be seen in cell-stained or Gram-stained preparations. An India ink preparation is useful in distinguishing between lymphocytes and cryptococci or Candida. Acid-fast bacilli will be found in appropriately stained samples. The monographs of den Hartog-Jager and the article of Bigner are older but still excellent references on CSF cytology. Special cell separation and immunostaining techniques permit the recognition of leukemia and lymphoma cell markers, glial fibrillary acidic protein, and other special cellular elements and antigens. These and other specific methods for the examination of cells in the CSF are discussed in the appropriate chapters.
In contrast to the high protein content of blood (5,500 to 8,000 mg/dL), that of the lumbar spinal fluid is 45 to 50 mg/dL or less in the adult. The protein content of CSF from the basal cisterns is 10 to 25 mg/dL and that from the ventricles is 5 to 15 mg/dL. This gradient may reflect the fact that CSF proteins leak from the blood plasma through capillary tight junctions, which form the blood–brain and blood–CSF barrier. The spinal fluid is an ultrafiltrate of blood made by the choroid plexus in the lateral and the fourth ventricles in a manner that is analogous to the formation of urine by the glomerulus. The amount of protein in the CSF would then be proportional to the length of time it is in contact with the blood–CSF barrier. Thus shortly after it is formed in the ventricles, the protein is low. More caudally in the basal cisterns, the protein is higher and in the lumbar subarachnoid space it is highest of all. In children, the protein concentration is somewhat lower at each level (<20 mg/dL in the lumbar subarachnoid space). Levels higher than normal indicate a pathologic process in or near the ependyma or meninges—in either the brain, spinal cord, or nerve roots—although the cause of modest elevations of the CSF protein, in the range of 75 mg/dL, frequently remains obscure.
As one would expect, bleeding into the ventricles or subarachnoid space results in spillage not only of RBCs but of serum proteins. If the serum protein concentrations are normal, the CSF protein should increase by about 1 mg per 1,000 RBCs provided that the same tube of CSF is used in determining the cell count and protein content. (The same holds for a traumatic puncture that allows seepage of venous blood into the CSF at the puncture site.) However, in the case of subarachnoid hemorrhage, caused by the irritating effect of hemolyzed RBC upon the leptomeninges, the CSF protein may be increased by many times this ratio.
The protein content of the CSF in bacterial meningitis, in which choroidal and meningeal perfusion are increased, often reaches 500 mg/dL or more. Viral infections induce a less intense and mainly lymphocytic reaction and a lesser elevation of protein—usually 50 to 100 mg/dL but sometimes up to 200 mg/dL; in some instances of viral meningitis and encephalitis the protein content is normal. Paraventricular tumors, by reducing the blood–CSF barrier, often raise the total protein to over 100 mg/dL. Protein values as high as 500 mg/dL are found in exceptional cases of the Guillain-Barré syndrome and chronic inflammatory demyelinating polyneuropathy. Values in the lumbar CSF of 1,000 mg/dL or more usually indicate a block to CSF flow; the fluid is then deeply yellow and clots readily because of the presence of fibrinogen; a combination called Froin syndrome. Partial CSF blocks by ruptured discs or tumor may elevate the protein to 100 to 200 mg/dL. Low CSF protein values are sometimes found in meningismus (a febrile illness with signs of meningeal irritation but normal CSF), in hyperthyroidism, or in conditions that produce low CSF pressure (e.g., after a recent LP as indicated in Chap. 30).
The quantitative partition of CSF proteins by electrophoretic and immunochemical methods demonstrate the presence of most of the serum proteins with a molecular weight of less than 150 to 200 kDa. The protein fractions that have been identified electrophoretically are prealbumin and albumin as well as alpha1, alpha2, beta1, beta2, and gamma globulin fraction, the last of these being accounted for mainly by immunoglobulins (the major immunoglobulin in normal CSF is IgG). The gamma globulin fraction in CSF is approximately 70 percent of that in serum. Table 2–2 gives the quantitative values of the different fractions. Immunoelectrophoretic methods have also demonstrated the presence of glycoproteins, ceruloplasmin, hemopexin, beta-amyloid and tau proteins. Large molecules—such as fibrinogen, IgM, and lipoproteins—are mostly excluded from the CSF unless generated there.
CEREBROSPINAL FLUID | SERUM | |
---|---|---|
Osmolarity | 295 mOsm/L | 295 mOsm/L |
Sodium | 138.0 mEq/L | 138.0 mEq/L |
Potassium | 2.8 mEq/L | 4.1 mEq/L |
Calcium | 2.1 mEq/L | 4.8 mEq/L |
Magnesium | 2.3 mEq/L | 1.9 mEq/L |
Chloride | 119 mEq/L | 101.0 mEq/L |
Bicarbonate | 23.0 mEq/L | 23.0 mEq/L |
Carbon dioxide tension | 48 mm Hg | 38 mm Hg (arterial) |
pH | 7.31–7.33 | 7.41 (arterial) |
Nonprotein nitrogen | 19.0 mg/dL | 27.0 mg/dL |
Ammonia | 30.0 g/dL | 70.0 g/dL |
Uric acid | 0.24 mg/dL | 5.5 mg/dL |
Urea | 4.7 mmol/L | 5.4 mmol/L |
Creatinine | 1.1 mg/dL | 1.8 mg/dL |
Phosphorus | 1.6 mg/dL | 4.0 mg/dL |
Total lipid | 1.5 mg/dL | 750.0 mg/dL |
Total cholesterol | 0.4 mg/dL | 180.0 mg/dL |
Cholesterol esters | 0.3 mg/dL | 126.0 mg/dL |
Glucose | 60 mg/dL | 90.0 mg/dL |
Lactate | 1.6 mEq/L | 1.0 mEq/L |
Total protein | 15–50 mg/dL | 6.5–8.4 g/100 dL |
Prealbumin | 1–7% | Trace |
Albumin | 49–73% | 56% |
Alpha1 globulin | 3–7% | 4% |
Alpha2 globulin | 6–13% | 10% |
Beta globulin (beta1 plus tau) | 9–19% | 12% |
Gamma globulin | 3–12% | 14% |
There are other notable differences between the protein fractions of CSF and plasma. The CSF always contains a prealbumin fraction and the plasma does not. Although derived from plasma, this fraction, for an unknown reason, concentrates in the CSF, and its level is greater in ventricular than in lumbar CSF, perhaps because of its concentration by choroidal cells. Also, tau (also identified as beta2-transferrin) is detected only in the CSF and not in other fluids; its concentration is also higher in the ventricular than in the spinal fluid. The concentration of tau protein and in particular the ratio of tau to beta-amyloid, has found use in the diagnosis of Alzheimer disease, as discussed in Chap. 39. At present only a few of these proteins are known to be associated with specific diseases of the nervous system. The most important is IgG, which may exceed 12 percent of the total CSF protein in diseases such as multiple sclerosis, neurosyphilis, subacute sclerosing panencephalitis and other chronic viral meningoencephalitides. The serum IgG is not correspondingly increased, which means that this immune globulin originates in (or perhaps is preferentially transported into) the nervous system. However, an elevation of serum gamma globulin—as occurs in cirrhosis, sarcoidosis, myxedema, and multiple myeloma—will be accompanied by a rise in the CSF globulin. Therefore, in patients with an elevated CSF gamma globulin, it is necessary to determine the electrophoretic pattern of the serum proteins as well. Certain qualitative changes in the CSF immunoglobulin pattern, particularly the demonstration of several discrete (oligoclonal) electrophoretic “bands”, each representing a specific immune globulin, and the ratio of IgG to total protein, are of special diagnostic importance in multiple sclerosis, as discussed in Chap. 36.
The albumin fraction of the CSF increases in a wide variety of central nervous system (CNS) and craniospinal nerve root diseases that increase the permeability of the blood–CSF barrier, but no specific clinical correlations can be drawn. Certain enzymes that originate in the brain, especially the brain-derived fraction of creatine kinase (CK-BB) but also enolase and neopterin, are found in the CSF after stroke, global ischemic hypoxia, or trauma, and have been used as markers of brain damage in experimental work. Other special markers such as elevation of the 14-3-3 protein, which has some diagnostic significance in prion disease, β2-microglobulin in meningeal lymphomatosis, neuron specific enolase in traumatic and other severe brain injuries, and alpha fetoprotein in embryonal tumors of the brain, may be useful in specialized circumstances.
The CSF glucose concentration is normally in the range of 45 to 80 mg/dL, i.e., about two-thirds of that in the blood (0.6 to 0.7 of serum concentrations). Higher levels parallel the blood glucose in this proportion; but with marked hyperglycemia, the ratio of CSF to blood glucose is reduced (0.5 to 0.6). With extremely low serum glucose, the ratio becomes higher, approximating 0.85. In general, CSF glucose values below 35 mg/dL are abnormal. After the intravenous injection of glucose, 2 to 4 h are required to reach equilibrium with the CSF; a similar delay follows the lowering of blood glucose. For these reasons, samples of CSF and blood for glucose determinations should ideally be drawn simultaneously in the fasting state or the serum should be obtained a few hours before the puncture but (this is often not practical). Low values of CSF glucose (hypoglycorrhachia) in the presence of pleocytosis usually indicate bacterial, tuberculous, or fungal meningitis, although similar reductions are observed in some patients with widespread neoplastic infiltration of the meninges and occasionally with sarcoidosis, subarachnoid hemorrhage (usually in the first week) and in chemically induced inflammation.
For a long time it was assumed that in meningitis the bacteria lowered the CSF glucose by their active metabolism, but the fact that the glucose remains at a subnormal level for 1 to 2 wk after effective treatment of the meningitis suggests that another mechanism is operative. Theoretically at least, an inhibition of the entry of glucose into the CSF, because of an impairment of the membrane transfer system, can be implicated. As a rule, viral infections of the meninges and brain do not lower the CSF glucose, although low glucose values have been reported in a small number of patients with mumps meningoencephalitis, and rarely in patients with herpes simplex and zoster infections. The almost invariable rise of CSF lactate in purulent meningitis probably suggests that some of the glucose is undergoing anaerobic glycolysis by polymorphonuclear leukocytes and by cells of the meninges and adjacent brain tissue.
CSF testing for cryptococcal surface antigen has become widely available as a rapid method if this infection is suspected. On occasion, a false-positive reaction is obtained in the presence of high titers of rheumatoid factor or antitreponemal antibodies, but otherwise the test is diagnostically more dependable than the formerly used India ink preparation. The nontreponemal antibody tests of the blood—Venereal Disease Research Laboratories (VDRL) slide flocculation test and rapid plasma reagin (RPR) agglutination test—can also be performed on the CSF. When positive, these tests are usually diagnostic of neurosyphilis, but false-positive reactions may occur with collagen diseases, malaria, and yaws, or with contamination of the CSF by seropositive blood. Tests that depend on the use of treponemal antigens, including the Treponema pallidum immobilization test and the fluorescent treponemal antibody test, are more specific and assist in the interpretation of false-positive RPR and VDRL reactions. The value of CSF examinations in the diagnosis and treatment of neurosyphilis is discussed in Chap. 32, but testing of CSF for treponemal antibodies is no longer routine. Serologic tests for the Lyme spirochete are useful in circumstances of suspected infection of the central nervous system with this agent.
The utility of serum serologic tests for viruses is limited by the time required to obtain results, but they are useful in determining retrospectively the source of meningitis or encephalitis. More rapid tests that use the polymerase chain reaction (PCR) in CSF, which amplifies viral DNA fragments, are now widely available for diagnosis, particularly for herpesviruses, cytomegalovirus, and JC virus. These tests are most useful in the first week of infection, when the virus is being reproduced and its genomic material is most prevalent; after this time, serologic techniques for viral infection are more sensitive. Amplification of DNA by PCR is particularly useful in the rapid detection of tubercle bacilli in the CSF, the conventional culture of which takes several weeks at best. Tests for the detection of 14-3-3- protein that reflects the presence of prion agents in the spinal fluid are available and may aid in the diagnosis of the spongiform encephalopathies, but the results have been erratic (Chap. 33).
The average osmolality of the CSF (295 mOsm/L) is identical to that of plasma. As the osmolality of the plasma is increased by the intravenous injection of hypertonic solutions such as mannitol or urea, there is a delay of up to several hours in the rise of osmolality of the CSF. It is during this period that the hyperosmolality of the blood maximally dehydrates the brain and decreases the volume of CSF. Table 2–2 lists the CSF and serum levels of sodium, potassium, calcium, and magnesium. Neurologic disease does not alter the CSF concentrations of these constituents in any characteristic way. The low CSF concentration of chloride that occurs in bacterial meningitis is not specific but a reflection of hypochloremia and, to a slight degree, of a greatly elevated CSF protein. Acid–base balance in the CSF is of interest in relation to metabolic acidosis and alkalosis but pH is not routinely measured. Normally, the pH of the CSF is approximately 7.31—i.e., somewhat lower than that of arterial blood, which is 7.41. The PCO2 in the CSF is in the range of 45 to 49 mm Hg—i.e., higher than in arterial blood (about 40 mm Hg). The bicarbonate levels of the two fluids are about the same, 23 mEq/L. The pH of the CSF is precisely regulated, and it tends to remain relatively unchanged even in the face of severe systemic acidosis and alkalosis. Acid–base changes in the lumbar CSF do not necessarily reflect the presence of similar changes in the brain, nor are the CSF data as accurate an index of the systemic changes as direct measurements of arterial blood gases.
The ammonia content of the CSF is one-third to one-half that of the arterial blood; it is increased in hepatic encephalopathy, the inherited hyperammonemias, and the Reye syndrome; the concentration corresponds roughly with the severity of the encephalopathy. The uric acid content of CSF is approximately 5 percent of that in serum and varies with changes in the serum level (high in gout, uremia, and meningitis, and low in Wilson disease). The urea concentration in the CSF is slightly lower than that in the serum; in uremia, it rises in parallel with that in the blood. An intravenous injection of urea raises the blood level immediately and the CSF level more slowly, exerting an osmotic dehydrating effect on the central nervous tissues and CSF. All 24 amino acids have been isolated from the CSF. The concentration of amino acids in the CSF is approximately one-third that in plasma. Elevations of glutamine are found in all of the portosystemic encephalopathies including hepatic coma and the Reye syndrome. Concentrations of phenylalanine, histidine, valine, leucine, isoleucine, tyrosine, and homocystine are increased in the corresponding aminoacidurias.
Many of the enzymes found in serum are known to rise in CSF under conditions of disease, usually in relation to a rise in the CSF protein. None of the enzyme changes has proved to be a specific indicator of neurologic disease with the possible exception of lactic dehydrogenase, especially isoenzymes 4 and 5, which are derived from granulocytes and are elevated in bacterial meningitis but not in aseptic or viral meningitis. Lactic dehydrogenase is also elevated in cases of meningeal tumor infiltration, particularly lymphoma, as is carcinoembryonic antigen; the latter, however, is not elevated in bacterial, viral, or fungal meningitis. As to lipids, the quantities in CSF are small and their measurement is difficult.
The catabolites of the catecholamines can be measured in the CSF. Homovanillic acid (HVA), the major catabolite of dopamine, and 5-hydroxyindoleacetic acid (5-HIAA), the major catabolite of serotonin, are normally present in the spinal fluid; both are five or six times higher in the ventricular than the lumbar CSF. The levels of both catabolites are reduced in patients with idiopathic and drug-induced parkinsonism.
Imaging Techniques of the Skull, Brain, and Spine
A century ago, Harvey Cushing introduced the use of plain x-ray films of the cranium as part of the study of the neurologic patient, but it is has become evident that the yield of useful information from this procedure is relatively small. Even in patients with head injury, where radiography of the skull would seem to be an optimal method of examination, a fracture is found in only 1 of 16 cases, at a cost of thousands of dollars per fracture and a small risk from radiation exposure. Nevertheless plain skull films do demonstrate fractures, changes in contour of the skull, bony erosions and hyperostoses, infection in paranasal sinuses and mastoids, and changes in the basal foramina. Plain films of the spine are able to demonstrate destructive lesions resulting from degenerative processes as well neoplastic, dysplastic, and infectious diseases. It also detects, fracture dislocations, spondylolistheses, and spinal instability, utilizing images acquired during flexion and extension maneuvers.
Refinements of radiographic techniques have greatly increased the yield of valuable information but without question the most important advances in neuroradiology have come about with the development of CT and MRI.
In this procedure, x-radiation is attenuated as it passes successively through the scalp, skull, CSF, cerebral gray and white matter, and blood vessels. The intensity of the exiting radiation relative to the incident radiation is measured, the data are integrated, and two-dimensional images are reconstructed by computer. This major achievement in methodology, attributed to Hounsfield and others, permitted the technologic advance from plain radiographs of the skull to reconstructed images of the cranium and its contents in any plane. The differing densities of bone, CSF, blood, and gray and white matter are distinguishable in the resulting picture with great clarity. One can see hemorrhage, infarcted, contused and edematous brain, abscess, and tumor tissue and also the precise size and position of the ventricles and midline structures. The radiation exposure is not significantly greater than that from plain skull films and comparable to a chest x-ray.
As illustrated in Fig. 2-1A-D, in transverse (axial) section of the brain, one sees the cortex and underlying subcortical white matter, the caudate and lenticular nuclei and the internal capsules and thalami. The position and width of all the major sulci and fissures can be measured, and the optic nerves and medial and lateral rectus muscles stand out clearly in the posterior parts of the orbit. The brainstem, cerebellum, and spinal cord are easily visible in the scan at appropriate levels. The scans are also useful in imaging parts of the body that surround peripheral nerves and plexuses, thereby demonstrating tumors, inflammatory lesions, and hematomas that involve these nerves. Intravenous administration of radio-opaque contrast can be used with CT to visualize regions where the blood-brain barrier has been disrupted from tumors, demyelination and infection.
Figure 2-1.
Normal axial CT scans of the brain, orbits, and skull base from a young healthy man. A
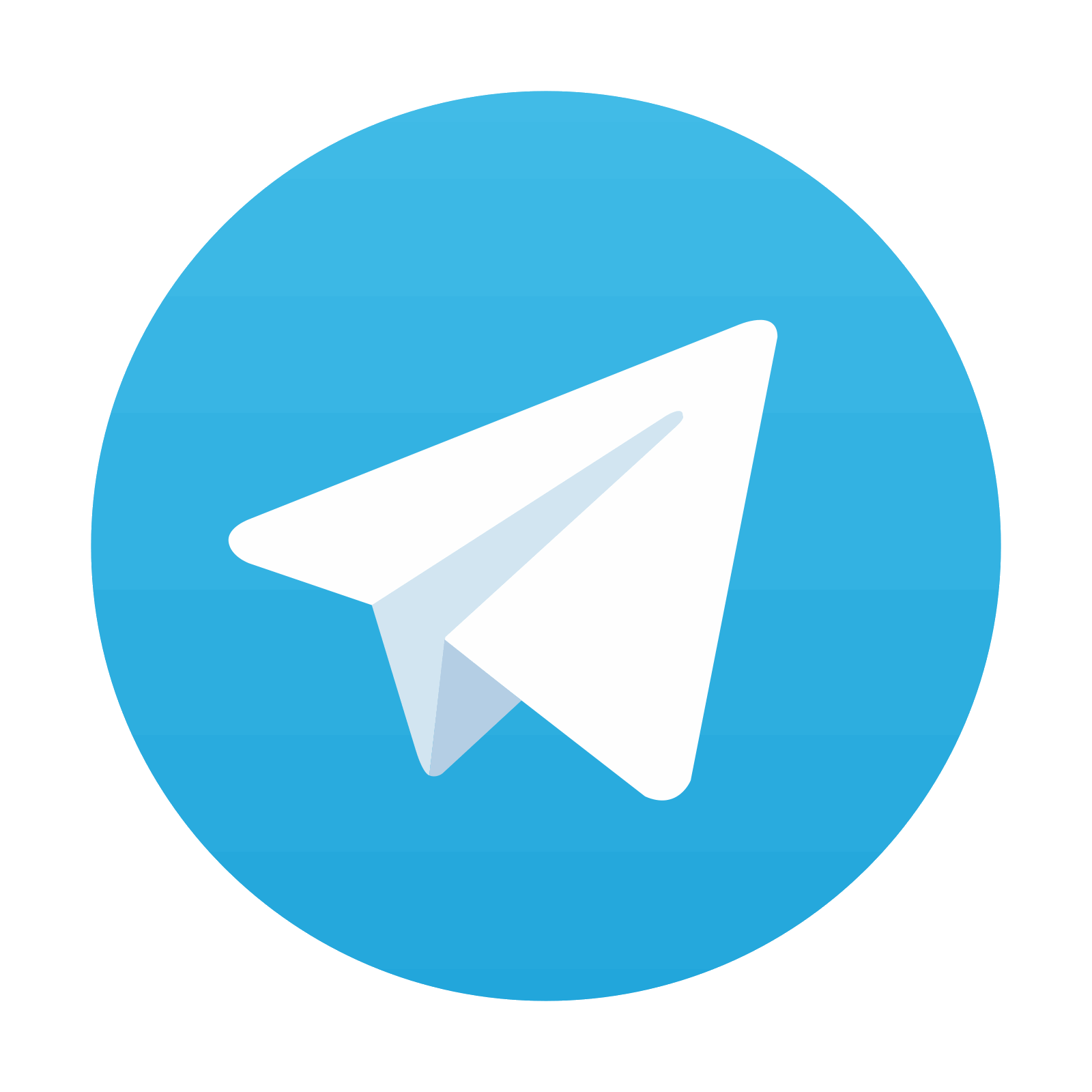
Stay updated, free articles. Join our Telegram channel
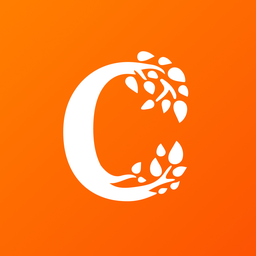
Full access? Get Clinical Tree
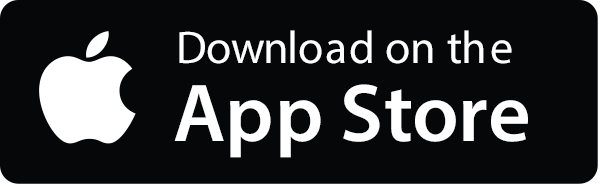
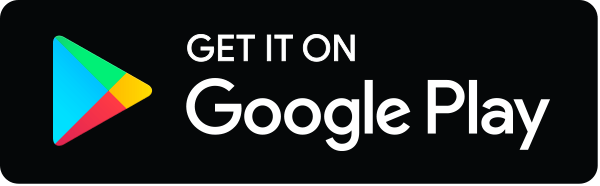
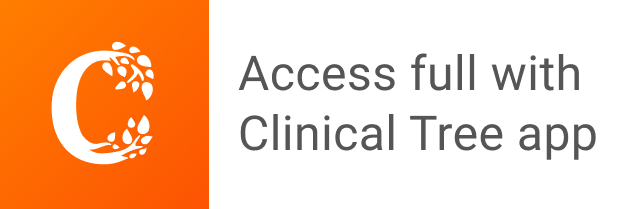