Definitions
Paralysis means loss of voluntary movement as a result of interruption of one of the motor pathways at any point from the cerebrum to the muscle fiber. A lesser degree of paralysis is spoken of as paresis. The word plegia comes from a Greek word meaning “to strike,” and the word palsy is from an old French word that has the same meaning as paralysis. One generally uses paralysis or plegia for severe or complete loss of motor function and paresis for partial loss.
The Lower Motor Neuron
Each spinal and cranial motor nerve cell, through the extensive arborization of the terminal part of its efferent fiber, comes into contact with only a few or up to 1,000 or more muscle fibers; together, the nerve cell, its axons, and the muscle fibers they subserve constitute the motor unit. All variations in the force, range, rate, and type of movement are determined by the number and size of motor units called into action and the frequency and sequence of firing of each motor unit. Weak movements involve relatively few small motor units; powerful movements recruit many more units that accumulate to an increasing size.
Within a few days after interruption of a motor nerve, the individual denervated muscle fibers begin to contract spontaneously. This isolated activity of individual muscle fibers is called fibrillation. Inability of the isolated fiber to maintain a stable membrane potential is the likely explanation. Fibrillation is so fine that it cannot be seen through the intact skin, but it can be recorded as a small, repetitive, short-duration potential in the electromyogram (EMG) (Chap. 45). When a motor neuron becomes diseased, it may manifest increased irritability, i.e., the axon is unstable and capable of spontaneous impulse generation, and all the muscle fibers that it controls may discharge sporadically, in isolation from other units. The result of contraction of one or several such motor units is a visible twitch of a muscle fascicle, or fasciculation, which appears in the EMG as a large spontaneous muscle action potential. Simultaneous or sequential spontaneous contractions of multiple motor units cause a rippling of muscle, a condition known as myokymia. If the motor neuron is destroyed, all the muscle fibers that it innervates undergo profound atrophy—termed denervation atrophy.
The motor nerve fibers of each ventral root intermingle with those of neighboring roots to form plexuses, and then the named peripheral nerves. Although the muscles are innervated roughly according to segments of the spinal cord, each large muscle is supplied by two or more roots. In contrast, a single peripheral nerve usually provides the complete motor innervation of a muscle or group of muscles. For this reason, paralysis caused by disease of the anterior horn cells or anterior roots has a different topographic pattern than paralysis following interruption of a peripheral nerve. These patterns follow the distribution shown in Table 46–1. For example, section of the L5 motor root causes paralysis of the extensors of the foot with a foot drop and weakness of inversion of the foot, whereas a lesion of the peroneal nerve also causes foot drop but does not affect the invertors of the foot that are also supplied by L5 but via the tibial nerve.
All motor activity, even the most elementary reflex type, requires the synchronous activity of many muscles. Analysis of a relatively simple movement, such as clenching the fist, conveys some idea of the complexity of the underlying neuromuscular arrangements. In this act the primary movement is a contraction of the flexor muscles of the fingers, the flexor digitorum sublimis and profundus, the flexor pollicis longus and brevis, and the abductor pollicis brevis. In the terminology of Beevor, these muscles act as agonists, or prime movers. For flexion to be smooth and forceful, the extensor muscles (antagonists) must relax at the same rate as the flexors contract (reciprocal innervation, or Sherrington law). The muscles that flex the fingers also flex the wrist. If it is desired that only the fingers flex, the extensors of the wrist must be brought into play to prevent its flexion; they are synergists. During this action of the hand, appropriate flexor and extensor muscles stabilize the wrist, elbow, and shoulder; muscles that accomplish this serve as fixators. The coordination of agonists, antagonists, synergists, and fixators is effected mainly by segmental spinal reflexes under the guidance of proprioceptive sensory stimuli. In general, the more delicate the movement, the more precise must be the coordination between agonist and antagonist muscles.
All voluntary ballistic (phasic) movements towards a target are accomplished by the activation of ensembles of motor neurons, large ones supplying large motor units and small ones, small motor units. The smaller ones are more efficiently activated by sensory afferents from muscle spindles, more tonically active, and more readily recruited in reflex activities, postural maintenance, walking, and running. The large motor units participate mainly in phasic movements, which are characterized by an initial burst of activity in the agonist muscles, then a burst in the antagonists, followed by a third smaller burst in the agonists. The strength of the initial agonist burst determines the speed and distance of the movement, but there is always the same triphasic pattern of agonist, antagonist, and agonist activity (Hallett et al). The basal ganglia and cerebellum set the pattern and timing of the muscle action in any projected motor performance. These points are discussed further in Chaps. 4 and 5.
Unlike the phasic movements just described, certain basic motor activities do not involve reciprocal innervation. In support of the body in an upright posture, when the legs must act as rigid pillars, and in shivering, agonists and antagonists contract simultaneously. Locomotion requires that the extensor pattern of reflex standing be inhibited and that the coordinated pattern of alternating stepping movements be substituted; the latter is accomplished by multisegmental spinal and brainstem reflexes, the so-called locomotor centers. Suprasegmental control of the axial and proximal limb musculature (antigravity postural mechanisms) is mediated primarily by the reticulospinal and vestibulospinal tracts and manipulatory movements of the distal extremity muscles, by the rubrospinal and corticospinal tracts. These aspects of motor function are elaborated further on.
Muscle stretch (tendon) reflex activity and muscle tone depend on the status of the large motor neurons of the anterior horn (the alpha motor neurons), the muscle spindles and their afferent fibers, and the small anterior horn cells (gamma neurons), whose axons terminate on the small intrafusal muscle fibers within the spindles. Each anterior horn cell has on its surface membrane approximately 10,000 receptive synaptic terminals. Some of these terminals are excitatory, others inhibitory; in combination, they determine the activity of the neuron. Beta motor neurons effect cocontraction of both spindle and nonspindle fibers, but the physiologic significance of this innervation is not fully understood. Some of the gamma motor neurons are tonically active at rest, keeping the intrafusal (nuclear chain) muscle fibers taut and sensitive to active and passive changes in muscle length.
A tap on a tendon stretches or perhaps causes vibration of the spindle and activates its nuclear bag fibers. Afferent projections from these fibers synapse directly with alpha motor neurons in the same and adjacent spinal segments; these neurons, in turn, send impulses to the skeletal muscle fibers, resulting in the familiar monosynaptic muscle contraction or monophasic (myotatic) stretch reflex, commonly referred to as the tendon reflex or “tendon jerk” (Fig. 3-1), more correctly called the muscle stretch or proprioceptive reflex. All this occurs within 25 ms of sudden stretch. The alpha neurons of antagonist muscles are simultaneously inhibited but through disynaptic rather than monosynaptic connections. This is accomplished in part by inhibitory interneurons (reciprocal inhibition), which also receive input from descending pathways. Renshaw cells also participate by providing negative feedback through inhibitory synapses of alpha motor neurons (recurrent inhibition).
Figure 3-1.
A. Patellar tendon reflex. Sensory fibers of the femoral nerve (spinal segments L2 and L3) mediate this myotatic reflex. The principal receptors are the muscle spindles, which respond to brisk stretching of the muscle effected by tapping the patellar tendon. Afferent fibers from muscle spindles are shown entering only the L3 spinal segment, while afferent fibers from the Golgi tendon organ are shown entering only the L2 spinal segment. In this monosynaptic reflex, afferent fibers entering spinal segments L2 and L3 and efferent fibers issuing from the anterior horn cells of these and lower levels complete the reflex arc. Motor fibers shown leaving the S2 spinal segment and passing to the hamstring muscles demonstrate the disynaptic pathway by which inhibitory influences are exerted upon an antagonistic muscle group during the reflex. B. The gamma loop is illustrated. Gamma efferent fibers (γ) pass to the polar portions of the muscle spindle. Contractions of the intrafusal fibers in the polar parts of the spindle stretch the nuclear bag region and thus cause an afferent impulse to be conducted centrally. The afferent fibers from the spindle synapse with many alpha motor neurons. Because the alpha motor neurons innervate extrafusal muscle fibers, excitation of the alpha motor neurons by spindle afferents causes a cocontraction of the muscle. In this way, both gamma and alpha fibers can simultaneously activate muscle contraction. Both alpha and gamma motor neurons are influenced by descending fiber systems from supraspinal levels. (Adapted by permission from Carpenter MB, Sutin J: Human Neuroanatomy, 8th ed. Baltimore, Williams & Wilkins, 1983.)
Thus the setting of the spindle fibers and the state of excitability of the alpha and gamma neurons (influenced greatly by descending fiber systems) determine the level of activity of the tendon reflexes and muscle tone (the responsiveness of muscle to stretch). Other mechanisms, of an inhibitory nature, involve the Golgi tendon organs, for which the stimulus is tension produced by active contraction of muscle. These encapsulated receptors, which lie in the tendinous and aponeurotic insertions of muscle, activate afferent fibers that end on internuncial cells, which, in turn, project to alpha motor neurons, thus forming a disynaptic reflex arc. Golgi tendon receptors are silent in relaxed muscle and during passive stretch; they serve, together with muscle spindles, to monitor or calibrate the length and force of muscle contraction under different conditions. They also play a role in naturally occurring limb movements, particularly in locomotion.
The alpha motor neurons of the medial parts of the anterior horn supply trunk or axial muscles, and neurons of the lateral parts supply the appendicular muscles. The largest neurons, in Rexed layer IX (see Fig. 8-1B), innervate large muscles with large motor units. Smaller anterior horn cells innervate small muscles and control more delicate movements, particularly those in the fingers and hand. Both groups of alpha neurons receive projections from neurons in the intermediate Rexed layers (V to VIII) and from propriospinal neurons in the fasciculi proprii of adjacent spinal segments (see Fig. 8-1B). All the facilitatory and inhibitory influences supplied by cutaneous and proprioceptive afferent and descending suprasegmental neurons are coordinated at segmental levels. For further details the reader may consult Burke and Lance and also Davidoff (1992).
There is considerable information concerning the pharmacology of motor neurons. The large neurons of the anterior horns of the spinal cord contain high concentrations of choline acetyltransferase and use acetylcholine as their transmitter at the neuromuscular junction. The main neurotransmitters of the descending corticospinal tract, in so far as can be determined in humans, are aspartate and glutamate. Glycine is the neurotransmitter released by Renshaw cells, which are responsible for recurrent inhibition, and by interneurons that mediate reciprocal inhibition during reflex action. Gamma-aminobutyric acid (GABA) serves as the inhibitory neurotransmitter of interneurons in the posterior horn. L-glutamate and L-aspartate are released by primary afferent terminals and interneurons and act specifically on excitatory amino acid receptors. There are also descending cholinergic, adrenergic, and dopaminergic axons, which play a less-well-defined role in reflex functions.
If all, or practically all, peripheral motor fibers supplying a muscle are interrupted, the voluntary, postural, and reflex movements of that muscle are abolished. The muscle becomes lax and soft and does not resist passive stretching, a condition known as flaccidity. Muscle tone—the slight resistance that normal relaxed muscle offers to passive movement—is reduced (hypotonia or atonia). The denervated muscle undergoes extreme atrophy, being reduced to 20 or 30 percent of its original bulk within 3 to 4 months. The reaction of the muscle to sudden stretch, as by tapping its tendon, is lost (areflexia). Damage restricted to only a portion of the motor fibers supplying the muscle results in partial paralysis, or paresis, and a proportionate diminution in the force and speed of contraction. The atrophy will be less and the tendon reflex reduced instead of lost. The electrodiagnosis of denervation depends upon finding fibrillations, fasciculations, and other abnormalities on needle electrode examination. However, some of these abnormalities do not appear until several days or a week or two after nerve injury (see Chap. 45).
Lower motor neuron (infranuclear) paralysis is the direct result of loss of function or destruction of anterior horn cells or their axons in anterior roots and nerves. The signs and symptoms vary according to the location of the lesion. In any individual case, the most important clinical question is whether sensory changes coexist. The combination of a flaccid, areflexic paralysis and sensory changes usually indicates involvement of mixed motor and sensory nerves or of both anterior and posterior roots. If sensory changes are absent, the lesion must be situated in the anterior gray matter of the spinal cord, in the anterior roots, in a purely motor branch of a peripheral nerve, or in motor axons alone (or in the muscle itself). At times it may be impossible to distinguish between nuclear (spinal) and anterior root (radicular) lesions.
Preserved and often heightened tendon reflexes and spasticity in muscles weakened by lesions of the corticospinal systems attest to the integrity of the spinal segments below the level of the lesion. However, acute and profound spinal cord lesions and, to a lesser extent, corticospinal lesions in the brainstem and cerebrum, may temporarily abolish spinal myotatic reflexes (“spinal shock”; see Chap. 44). This is caused by the interruption of descending tonic excitatory impulses, which normally maintain a sufficient level of excitation in spinal motor neurons to permit the peripheral activation of segmental reflexes. The attenuation of spinal shock by opiate antagonists, such as naloxone, suggests that the phenomenon is at least in part mediated by the release of previously stored endogenous opiates from the distal terminals of neurons in the periaqueductal gray matter. Once the stored opiates are depleted, the presynaptic inhibition of motor neurons ceases, heralding the end of spinal shock and the beginning of the period of spasticity.
The Upper Motor Neuron
The terms pyramidal, corticospinal, and upper motor neuron are often used interchangeably, although they are not altogether synonymous. The pyramidal tract, strictly speaking, designates only those fibers that course longitudinally in the pyramid of the medulla oblongata. Of all the fiber bundles in the brain, the pyramidal tract has been known for the longest time, the first accurate description having been given by Türck in 1851. It descends from the cerebral cortex; traverses the subcortical white matter (corona radiata), internal capsule, cerebral peduncle, basis pontis (ventral pons), and pyramid of the upper medulla; decussates in the lower medulla; and continues its caudal course in the lateral funiculus (column) of the spinal cord—hence the alternative name corticospinal tract. This is the only direct long-fiber connection between the cerebral cortex and the spinal cord (Fig. 3-2). The indirect pathways through which the cortex influences spinal motor neurons are the rubrospinal, reticulospinal, vestibulospinal, and tectospinal; these tracts do not run in the pyramid. All of these pathways, direct and indirect, are embraced by the term upper motor neuron or supranuclear, meaning above the anterior horn cells.
A major source of confusion about the pyramidal tract stems from the traditional view, formulated at the turn of the 20th century, that it originates entirely from the large motor cells of Betz in the fifth layer of the precentral convolution (the primary motor cortex, or area 4 of Brodmann1) (Figs. 3-3 and 22-1). However, there are only some 25,000 to 35,000 Betz cells, whereas the medullary pyramid contains about 1 million axons (Lassek). Thus the pyramidal tract contains many fibers that arise from cortical neurons other than Betz cells, particularly in Brodmann areas 4 and 6 (the frontal cortex immediately rostral to area 4, including the medial portion of the superior frontal gyrus, i.e., the supplementary motor area); in the primary somatosensory cortex (Brodmann areas 3, 1, and 2); and in the superior parietal lobule (areas 5 and 7). Data concerning the origin of the pyramidal tract in humans are scanty, but in the monkey, by counting the pyramidal axons that remained after cortical excisions and long survival periods, Russell and DeMyer found that 40 percent of the descending axons arose in the parietal lobe, 31 percent in motor area 4, and the remaining 29 percent in premotor area 6. Studies of retrograde transport of tracer substance in the monkey have confirmed these findings.
Figure 3-3.
Lateral (A) and medial (B) surfaces of the human cerebral hemispheres, showing the areas of excitable cortex, i.e., areas, numbered according to the scheme of Brodmann. (Reprinted with permission from House EL, Pansky B: A Functional Approach to Neuroanatomy, 2nd ed. New York, McGraw-Hill, 1967.) See also Fig. 22-1.
Fibers from the motor and premotor cortices (Brodmann areas 4 and 6, Fig. 22-1), supplementary motor cortex, and portions of parietal cortex (areas 1, 3, 5, and 7) converge in the corona radiata and descend through the posterior limb of the internal capsule, basis pedunculi, basis pontis, and medulla. As the corticospinal tracts descend in the cerebrum and brainstem, they send collaterals to the striatum, thalamus, red nucleus, cerebellum, and reticular formations. Accompanying the corticospinal tracts in the brainstem are the corticobulbar tracts, which are distributed to motor nuclei of the cranial nerves ipsilaterally and contralaterally (Fig. 3-2). It has been possible to trace the direct projection of axons of cortical neurons to the trigeminal, facial, ambiguus, and hypoglossal nuclei (Iwatsubo et al). No axons were seen to terminate directly in the oculomotor, trochlear, abducens, or vagal nuclei. Insofar as the corticobulbar and corticospinal fibers have a similar origin and the motor nuclei of the brainstem are the homologues of the motor neurons of the spinal cord, the term upper motor neurons may suitably be applied to both these systems of fibers.
The corticospinal tracts decussate at the lower end of the medulla, although some of their fibers may cross above this level. The fibers destined for the upper limb neurons cross first (more rostrally). The proportion of crossed and uncrossed fibers varies to some extent from one person to another. About 75 to 80 percent of the fibers cross and the remaining fibers descend ipsilaterally, mostly in the uncrossed ventral corticospinal tract. In exceptional cases, these tracts cross completely; equally rarely, they remain uncrossed. These variations are probably of functional significance in determining the amount of neurologic deficit that results from a unilateral lesion such as capsular infarction. A few well-studied cases are found, such as the one described by Terakawa and colleagues, of acute stroke of the cerebral hemisphere causing hemiplegia on the same side. Also, Yakovlev found 3 instances of completely uncrossed pyramids among 130 autopsies of mentally retarded neonates but considering the maldevelopment of these brains, the finding may not be surprising.
The corticospinal tract is phylogenetically relatively new, being found only in mammals, which probably accounts for its variability between individuals as compared to the older vestibulospinal, rubrospinal and reticulospinalparapyramidal systems, which are invariant among persons. Uncrossed fibers in the corticospinal tract account for mirror movements that are seen during efforts at fine motor tasks, particularly in children, and also in some disorders of the nervous system such as the Klippel-Feil syndrome and the Kallmann syndrome. For a more complete discussion of the crossing of the various tracts of the nervous system, the reader is referred to the review by Vulliemoz, Raineteau, and Jabaudon.
Beyond their decussation, the corticospinal pathways descend as well-defined bundles in the anterior and posterolateral columns of white matter (funiculi) of the spinal cord (Fig. 3-2). The course of the noncorticospinal motor pathways (vestibulospinal, reticulospinal, and descending propriospinal) have been traced in humans by Nathan and his colleagues. The lateral vestibulospinal tract lies at the periphery of the cord, where it occupies the most anterolateral portion of the anterior funiculus. The medial vestibulospinal fibers mingle with those of the medial longitudinal fasciculus. Reticulospinal fibers are less compact; they descend bilaterally, and most of them come to lie just anterior to the lateral corticospinal tract. The descending propriospinal pathway consists of a series of short fibers (one or two segments long) lying next to the gray matter.
The somatotopic organization of the corticospinal system is of importance in clinical work, especially in relation to certain stroke syndromes. As the descending axons subserving limb and facial movements emerge from the cortical motor strip, they maintain the anatomic organization of the overlying cortex; therefore a discrete cortical–subcortical lesion will result in a restricted weakness of the hand and arm or the foot and leg. More caudally, the descending motor fibers converge and are collected in the posterior limb of the internal capsule, so that even a small lesion there will cause a “pure motor hemiplegia,” in which the face, arm, hand, leg, and foot are affected to more or less the same degree (see Lacunar syndromes in Chap. 34). The axons subserving facial movement are situated rostrally in the posterior limb of the capsule, those for hand and arm in the central portion and those for the foot and leg, caudally (as detailed by Brodal).
This topographic distribution is maintained in the cerebral peduncle, where the corticospinal fibers occupy approximately the middle of the peduncle, the fibers destined to innervate the facial nuclei lying most medially. More caudally, in the basis pontis (base, or ventral part of the pons), the descending motor tracts separate into bundles that are interspersed with masses of pontocerebellar neurons and their cerebellipetal fibers. A degree of somatotopic organization can be recognized here as well, exemplified by selective weakness of the face and hand with dysarthria, or of the leg, which may occur with pontine lacunar infarctions. Anatomic studies in nonhuman primates indicate that arm–leg distribution of fibers in the rostral pons is much the same as in the cerebral peduncle; in the caudal pons, this distinction is less-well defined. In humans, a lack of systematic anatomic study leaves the precise somatotopic organization of corticospinal fibers in the pons less certain. Restricted pontine lesions may cause a pure motor hemiplegia that is indistinguishable from the syndrome of the internal capsule. However, a study conducted by Marx and colleagues using sophisticated MRI mapping techniques of patients with hemiplegia from brainstem lesions suggests that the usual somatotopic organization breaks down in the base of the pons, and there is a concentration of fibers innervating proximal muscles lying more dorsally and those exciting distal parts of the limbs, more ventrally.
Another point of uncertainty has been the existence and course of fibers that descend through the lower pons and upper medulla and then ascend again to innervate the facial motor nucleus on the opposite side. Such a connection must exist to explain occasional instances of facial palsy from brainstem lesions caudal to the midpons. A discussion of the various hypothesized sites of this pathway, including a recurrent tract (Pick bundle), can be found in the report by Terao and colleagues. They conclude from imaging studies that corticobulbar fibers destined for the facial nucleus descend in the ventromedial pons to the level of the upper medulla, where they decussate and then ascend again; but there is considerable variation between individuals in this configuration.
The descending pontine bundles, now devoid of their corticopontine fibers, reunite to form the medullary pyramid. The brachial–crural pattern may persist in the pyramids and is certainly reconstituted in the lateral columns of the spinal cord (Fig. 8-3), but it should be emphasized that the topographic separation of motor fibers at cervical, thoracic, lumbar, and sacral levels is not as discrete as usually shown in schematic diagrams of the spinal cord.
The corticospinal tracts and other upper motor neurons terminate mainly in relation to nerve cells in the intermediate zone of spinal gray matter (internuncial neurons), from which motor impulses are then transmitted to the anterior horn cells. Only 10 to 20 percent of corticospinal fibers (presumably the thick, rapidly conducting axons derived from Betz cells) establish direct synaptic connections with the large motor neurons of the anterior horns.
The motor area of the cerebral cortex is defined physiologically as the region of electrically excitable cortex from which isolated movements can be evoked by stimuli of minimal intensity. The muscle groups of the contralateral face, arm, trunk, and leg are represented in the primary motor cortex (area 4 in Fig. 3-3), those of the face being in the most inferior part of the precentralgyrus on the lateral surface of the cerebral hemisphere and those of the leg in the paracentral lobule on the medial surface of the cerebral hemisphere. The parts of the body capable of the most delicate movements have, in general, the largest cortical representation, as displayed in the motor homunculus (“little man,” a term first suggested by Wilder Penfield) shown in Fig. 3-4.
Area 6, the premotor area, is also electrically excitable but requires more intense stimuli than area 4 to evoke movements. Stimulation of its caudal aspect (area 6a) produces responses that are similar to those elicited from area 4. These responses are probably produced by transmission of impulses from all of area 6a to area 4 (as they cannot be obtained after ablation of area 4). Stimulation of the rostral premotor area (area 6a) elicits more general movement patterns, predominantly of proximal limb musculature. The latter movements are effected via pathways other than those derived from area 4 (hence, “parapyramidal”). Very strong stimuli elicit movements from a wide area of premotor frontal and parietal cortex, and the same movements may be obtained from several widely separated points. From this it may be assumed, as Ash and Georgopoulus point out, that the premotor cortex includes several anatomically distinct subregions with different afferent and efferent connections. In general, it may be said that the motor–premotor cortex is capable of synthesizing agonist actions into an almost infinite variety of finely graded, highly differentiated patterns. These are directed by visual (area 7) and tactile (area 5) sensory information and supported by appropriate postural mechanisms.
The supplementary motor area is the most anterior portion of area 6 on the medial surface of the cerebral hemisphere (area 6a in Fig. 3-3B). Stimulation of this area may induce relatively gross ipsilateral or contralateral movements, bilateral tonic contractions of the limbs, contraversive movements of the head and eyes with tonic contraction of the contralateral arm, and sometimes inhibition of voluntary motor activity and vocal arrest.
Precisely how the motor cortex controls movements is still a controversial matter. The traditional view, based on the interpretations of Hughlings Jackson and of Sherrington, has been that the motor cortex is organized not in terms of individual muscles but of movements, i.e., the coordinated contraction of groups of muscles. Jackson visualized a widely overlapping representation of muscle groups in the cerebral cortex on the basis of his observation that a patient could recover the use of a limb following destruction of the limb area as defined by cortical stimulation. This view was supported by Sherrington’s observations that stimulation of the cortical surface activated not solitary muscles but a combination of muscles, and always in a reciprocal fashion—i.e., in a manner that maintained the expected relationship between agonists and antagonists. He also noted the inconstancy of stimulatory effects; the stimulation of a given cortical point that initiated flexion of a part on one occasion might initiate extension on another.
These interpretations must be viewed with circumspection, as must all observations based on the electrical stimulation of the surface of the cortex. It has been shown that to stimulate motor cells from the surface, the electric current has to penetrate the cortex to layer V, where these neurons are located, inevitably activating a large number of other cortical neurons. The elegant experiments of Asanuma and of Evarts and his colleagues, who stimulated the depths of the cortex with microelectrodes, demonstrated the existence of discrete zones of efferent neurons that control the contraction of individual muscles; moreover, the continued stimulation of a given efferent zone often facilitated rather than inhibited the contraction of the antagonists. These investigators have also shown that cells in the efferent zone receive afferent impulses from the particular muscle to which the efferent neurons project. When the effects of many stimulations at various depths were correlated with the exact sites of each penetration, cells that projected to a particular pool of spinal motor neurons were found to be arranged in radially aligned columns approximately 1 mm in diameter.
The columnar arrangement of cells in the sensorimotor cortex had been appreciated for many years; the wealth of radial interconnections between the cells in these columns led Lorente de Nó to suggest that these “vertical chains” of cells were the elementary functional units of the cortex. This notion received strong support from Mountcastle’s observations that all the neurons in a column receive impulses of the same sensory modality, from the same part of the body. It is still not entirely clear whether the columns contribute to a movement as units or whether individual cells within many columns are selectively activated. Both Henneman and Asanuma summarized the evidence for these disparate views.
Evarts and his colleagues also elucidated the role of cortical motor neurons in sensory evoked or planned movement. Using single-cell recording techniques, they showed that pyramidal cells fire about 60 ms prior to the onset of a movement, in a sequence determined by the required pattern and force of the movement. But other, more complex properties of the pyramidal cells were also noted. Some of them received a somatosensory input transcortically from the parietal lobe (areas 3, 1, and 2), which could be turned on or off or gated according to whether the movement was to be controlled, i.e., guided, by sensory input. Many neurons of the supplementary and premotor cortices were activated before a planned movement. Thus pyramidal (area 4) motor neurons were prepared for the oncoming activation by impulses from the parietal, prefrontal, premotor, and auditory and visual areas of the cortex. This preparatory “set signal” could occur in the absence of any activity in the spinal cord and muscles. The source of the activation signal was found to be mainly in the supplementary motor cortex, which appears to be under the direct influence of the “readiness stimuli” (Bereitschaft potential) reaching it from the prefrontal areas for planned movements and from the posterior parietal cortex for motor activities initiated by sensory perceptions. There are also fibers that reach the motor area from the limbic system, presumably subserving motivation and attention. Roland has used functional cerebral blood flow measurements to follow these neural events.
Thus the prefrontal cortex, supplementary motor cortex, premotor cortex, and motor cortex are all responsive to afferent stimuli and are involved prior to, and in coordinated fashion with, a complex movement. As remarked later on, the striatopallidum and cerebellum, which project to these cortical areas, are also activated prior to or concurrently with the discharge of corticospinal neurons (see Thach and Montgomery for a critical review of the physiologic data).
This has been studied in the monkey by interrupting the descending motor pathways in the medulla and more rostral parts of the brainstem and tracing the distribution of the degenerating elements in the spinal gray matter. On the basis of such experiments and other physiologic data, Lawrence and Kuypers proposed that the functional organization of the descending cortical and subcortical pathways is determined more by their patterns of termination and the motor capacities of the internuncial neurons upon which they terminate than by the location of their cells of origin. Three groups of motor fibers were distinguished according to their differential terminal distribution: (1) The corticospinal and corticobulbar tracts, which project to all levels of the spinal cord and brainstem, terminating diffusely throughout the nucleus proprius of the dorsal horn and the intermediate zone. A portion of these connect directly with the large motor neurons that innervate the muscles of the fingers, face, and tongue; this system provides the capacity for a high degree of fractionation of movements, as exemplified by independent finger movements.
As alluded to above, a large fraction of the fibers in the corticospinal originate from the sensory cortex and appear to function in the modulation of movement by afferent neurons. (2) A ventromedial pathway, which arises in the tectum (tectospinal tract), vestibular nuclei (vestibulospinal tract), and pontine and medullary reticular cells (reticulospinal tract
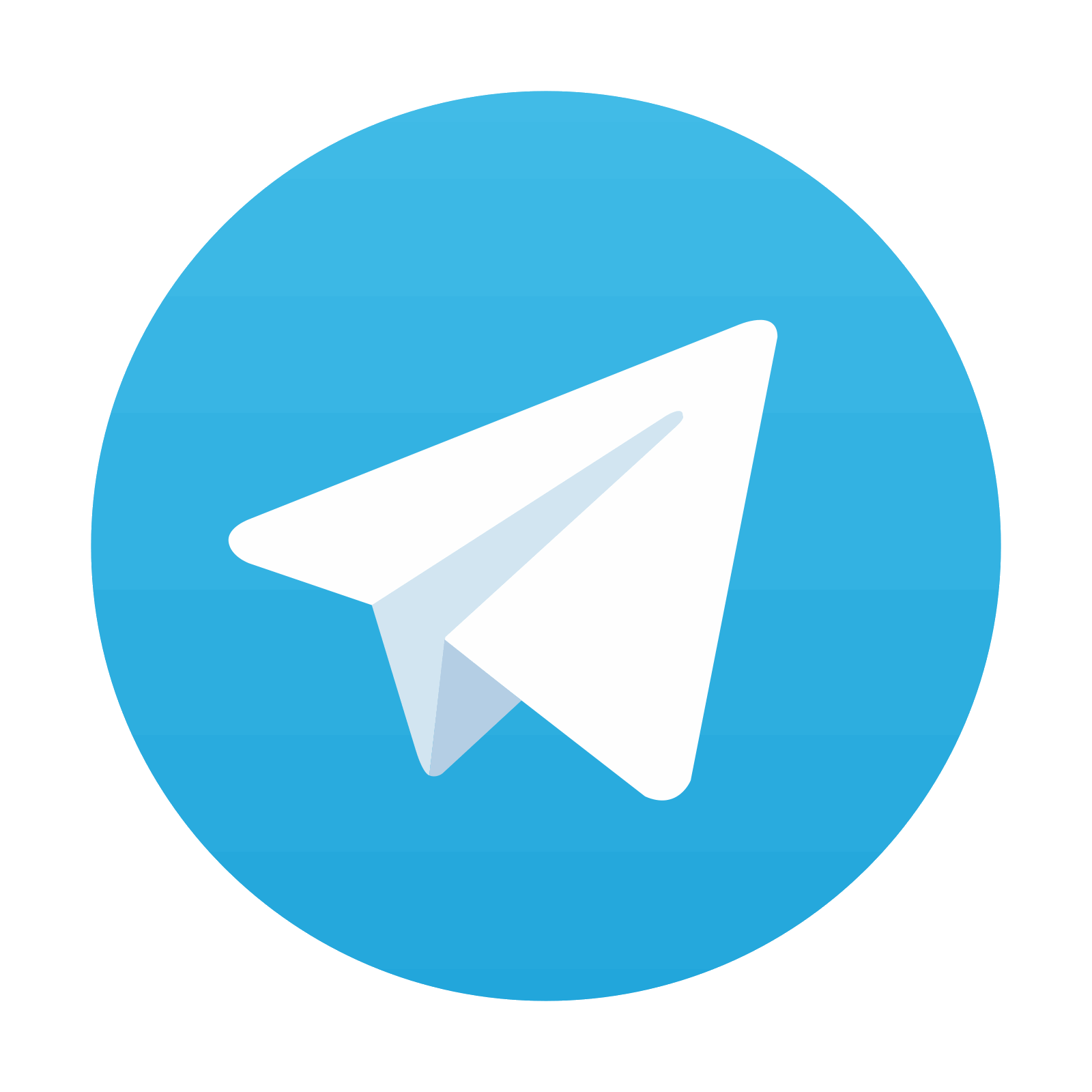
Stay updated, free articles. Join our Telegram channel
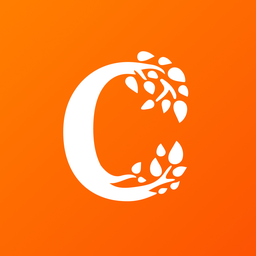
Full access? Get Clinical Tree
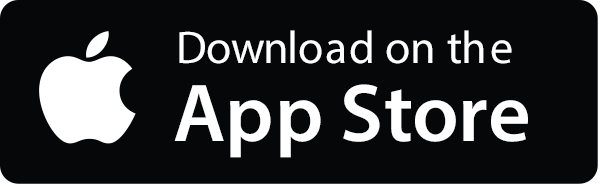
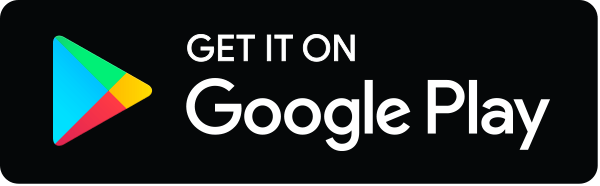
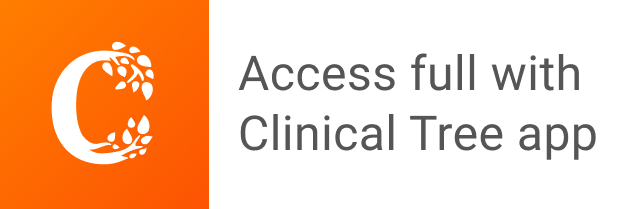