Chapter 32B Clinical Neurophysiology
Clinical Electromyography
Clinical electromyography is a distinct medical discipline that plays a pivotal role in the diagnosis of neuromuscular disorders (Katirji et al., 2002). The designations clinical electromyography, electrodiagnostic examination, and electroneuromyography are used interchangeably to encompass the electrophysiological study of nerve and muscle; the terms needle electromyography or needle electrode examination are reserved for the specific testing that involves needle electrode evaluation of muscle. Although many still refer to all such testing as simply electromyography (EMG), use of the word without a descriptor is discouraged because it can be confusing, often implying only the needle electrode part of the evaluation. For clarity, this chapter will refer to clinical EMG or needle EMG in the context of the discussion.
The clinical EMG examination is an important diagnostic tool that helps localize a neuromuscular problem at the motor neurons, nerve roots, peripheral nerves, neuromuscular junction, or muscle. It also helps establish the underlying process in these disorders and assess their management and prognosis. Electrodiagnostic testing provides the most valuable diagnostic information when the clinical assessment suggests a short list of differential diagnoses. The clinician should perform a detailed or focused neurological examination before referring the patient for a clinical EMG, which in turn serves as an independent procedure to provide an objective assessment of the peripheral nervous system (PNS) (Katirji, 2002). Patients with complex clinical pictures are best served by a neurological consultation prior to ordering electrodiagnostic testing.
The clinical EMG examination is composed of two main tests: nerve conduction studies (NCS) and needle EMG. These tests complement each other, and both often are necessary for a definite diagnosis. Additional electrodiagnostic procedures include assessment of F waves, H reflexes, and blink reflexes; repetitive nerve stimulation; and single-fiber EMG. A focused history and examination will help the electromyographer design the most appropriate electrodiagnostic study (Preston and Shapiro, 2005). The electromyographer must be proficient in using modern electrodiagnostic equipment and applying electrodiagnostic techniques, know the normal values for commonly and uncommonly examined nerve conduction studies and for motor unit action potentials (MUAPs) in different muscles, and be familiar with the specific and nonspecific electrodiagnostic findings in different neuromuscular disorders.
Nerve Conduction Studies
Motor Nerve Conduction Studies
The nerve usually is stimulated, whenever technically feasible, at two or more points along its course. Shorter nerves such as the axillary, femoral, and facial nerves are stimulated at only one point, because the more proximal portions of the nerves are inaccessible. Otherwise, the nerve typically is stimulated distally near the recording electrode and more proximally to evaluate one or more proximal segments. Motor NCS evaluate several measurements (Fig. 32B.1):
CMAP amplitude: The usual measure of amplitude is from baseline to negative peak and expressed in millivolts. When recorded with surface electrodes, CMAP amplitude is a semiquantitative measure of the number of axons conducting between the stimulating and recording points. CMAP amplitude also depends on the relative conduction speed of the axons, the integrity of the neuromuscular junctions, and the number of muscle fibers that are able to generate action potentials.
CMAP duration: This measurement usually is the duration of the negative phase of the evoked potential and expressed in milliseconds. It is a function of the conduction rates of the various axons forming the examined nerve and the distance between the stimulation and recording electrodes. The CMAP generated from proximal stimulation is slightly longer in duration and of lower amplitude than that obtained from distal stimulation, as a result of temporal dispersion and phase cancellation (see forthcoming section).
CMAP area: This usually is limited to the negative phase area under the waveform and shows linear correlation with the product of amplitude and duration. Measurement is in millivolts per millisecond and requires electronic integration using computerized equipment. The ability to measure CMAP area has practically replaced the need to measure its duration.
Latencies: Latency is the time interval between nerve stimulation (shock artifact) and the CMAP onset. Expression of latency is in milliseconds and reflects the conduction rate of the fastest-conducting axon. Whenever technically possible, the nerve is stimulated at two points: a distal point near the recording site and a more proximal point; the measures obtained are the distal latency and proximal latency, respectively. Both latencies depend mostly on the length of the nerve segment and, to a much lesser extent, on neuromuscular transmission time and propagation time along the muscle membrane.
Conduction velocity: This is a computed measurement of the speed of conduction expressed in meters per second. Measurement of conduction velocity allows comparison of the speed of conduction of the fastest fibers between different nerves and subjects, irrespective of the length of the nerve. The calculation requires measurement of the length of the nerve segment between distal and proximal stimulation sites. Measuring the surface distance along the course of the nerve estimates the nerve length; it should be more than 10 cm to improve the accuracy of surface measurement.
As with latencies, motor conduction velocity measures the speed of conduction of the fastest axon. In contrast with motor latency, however, motor nerve conduction velocity is a pure nerve conduction time, because neuromuscular transmission time and muscle fiber propagation time are common to both stimulation sites, and the latency difference between two points is the time required for the nerve impulse to travel from one stimulus point to the other.
Sensory Nerve Conduction Studies
SNAPs may be obtained by several methods: (1) stimulating and recording a pure sensory nerve (such as the sural and radial sensory nerves); (2) stimulating a mixed nerve while recording distally over a cutaneous branch (such as the antidromic median and ulnar sensory responses), or (3) stimulating a distal cutaneous branch while recording over a proximal mixed nerve (such as in orthodromic median and ulnar sensory studies). Similar to their motor counterparts, sensory NCS record several measurements (Fig. 32B.2):
SNAP amplitude: This semiquantitatively measures the number of sensory axons that conduct between the stimulation and recording sites. The calculation is from the baseline to negative peak or from negative peak to positive peak, and expressed in microvolts. SNAP duration and area may be measured but are not useful because of significant temporal dispersion and phase cancellation (see later discussion).
Latencies: Sensory distal latencies are measured (in milliseconds) from the stimulus artifact to the peak of the negative phase (peak latency) or from the stimulus artifact to the onset of the SNAP (onset latency). A large shock artifact, a noisy background, or a wavy baseline may obscure onset latency. Although peak latency does not reflect the fastest-conducting sensory fibers, it is easily defined and more precise than onset latency.
Conduction velocity: This requires stimulation at a single site only because the latency consists of just the nerve conduction time from the stimulus point to the recording electrode. As with motor velocity, the calculation may be done using both distal and proximal stimulations. Only onset latencies (not peak latencies) are useful to calculate velocities to assess the speed of the fastest-conducting fibers.
Segmental Stimulation in Short Increments
The inching (or actually “centimetering”) technique is particularly useful in assessing nerve conduction in patients with carpal tunnel syndrome or an ulnar neuropathy at the elbow or wrist (McIntosh et al., 1998). For example, stimulation of a normal median nerve in 1-cm increments across the wrist results in latency changes of approximately 0.16 to 0.21 msec/cm from midpalm to distal forearm (Fig. 32B.3). A sharply localized latency increase across a 1-cm segment indicates a focal abnormality of the median nerve (Fig. 32B.4). An abrupt change in waveform usually accompanies the latency increase across the site of compression.
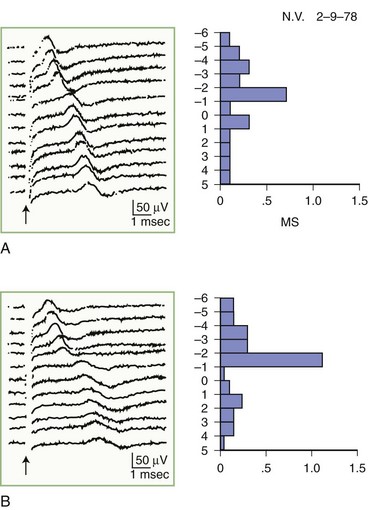
Fig. 32B.4 Sensory nerve action potentials in a patient with bilateral carpal tunnel syndrome (see also Fig. 35B.3 for settings). A sharply localized slowing was found from point −2 to point −1 in both hands, with a latency change measuring 0.7 msec on the left (A) and 1.1 msec on the right (B), compared with the other segments with normal latency changes of approximately 0.16 to 0.21 msec. Note also a distinct change in waveform of the sensory potential at the point of localized conduction delay.
(Reprinted with permission of the author and publisher from Kimura, J., 1979. The carpal tunnel syndrome: localization of conduction abnormalities within the distal segment of the median nerve. Brain 102, 619-635. By permission of Oxford University Press, Inc.)
Physiological Variability and Common Sources of Error
The major pitfalls in NCS usually involve technical errors in the stimulating or recording system (Kimura, 1997). Common errors include large stimulus artifact, increased electrode noise, submaximal stimulation, co-stimulation of adjacent nerve not under study, eliciting an unwanted potential from distant muscles, recording or reference electrode misplacement, and errors in measurement of nerve length and conduction time. Other errors are attributable to intertrial and physiological variability, which include the effects of temperature, age, the length of studied nerve, anomalous innervation, and temporal dispersion.
Temperature
Nerve impulse propagation slows by 2.4 m/sec, or approximately 5%, per degree centigrade from 38°C to 29°C of body temperature. Also, cooling results in a higher CMAP and SNAP amplitude and longer response duration, probably because of accelerated and slowed sodium channel inactivation (Rutkove et al., 1997). Therefore, a CMAP or SNAP with high amplitude and slow distal latency or conduction velocity should raise the suspicion of a cool limb. To reduce this type of variability, a plate thermistor measures skin temperature. This measurement correlates linearly with the subcutaneous and intramuscular temperatures. If the skin temperature falls below 33°C, warm the limbs by immersion in warm water or by application of warming packs or a hydrocollator. Adding 5% of the calculated conduction velocity for each degree below 33°C theoretically normalizes the result. The use of such conversion factors is based on evidence obtained in healthy persons, however, and may not be applicable in patients with abnormal nerves.
Anomalies
Martin-Gruber Anastomosis
In the Martin-Gruber anastomosis, anomalous fibers cross from the median to the ulnar nerve in the forearm. The communicating branches usually consist of motor axons supplying the ulnar innervated intrinsic hand muscles, particularly the first dorsal interosseous muscle, the hypothenar muscles (abductor digiti minimi), and the thenar muscles (adductor pollicis, deep head of flexor pollicis brevis), or a combination of these muscles. The Martin-Gruber anastomosis occurs in approximately 15% to 20% of the population and sometimes is bilateral. This anomaly manifests as a drop in the ulnar CMAP amplitude between distal and proximal stimulation sites (simulating the appearance of conduction block on ulnar NCS recording from the abductor digiti minimi or first dorsal interosseous). With distal stimulation (at the wrist), the CMAP reflects all ulnar motor fibers, whereas proximal stimulation activates only the uncrossed fibers, which are fewer in number. This anomaly can be confirmed by median nerve stimulation at the elbow, which evokes a small CMAP from the abductor digiti minimi or first dorsal interosseous, which is not present on median nerve stimulation at the wrist. When anomalous fibers innervate the thenar muscles, stimulation of the median nerve at the elbow activates the nerve and the crossing ulnar fibers, resulting in a large CMAP, often with an initial positivity caused by volume conduction of action potential from the ulnar thenar muscles to the median thenar muscles. By contrast, distal median nerve stimulation evokes a smaller thenar CMAP without the positive dip because the crossed fibers are not present at the wrist. In addition, the median nerve conduction velocity in the forearm is spuriously fast, particularly in the presence of carpal tunnel syndrome because the CMAP onset represents a different population of fibers at the wrist than at the elbow. Collision studies obtain an accurate conduction velocity by using action potentials of the crossed fibers (Sander et al., 1997).
Accessory Deep Peroneal Nerve
About 20% to 30% of subjects have an anomalous deep peroneal nerve. It is a branch of the superficial peroneal nerve and usually arises as a continuation of the muscular branch that innervates the peroneus longus and brevis muscles. It passes behind the lateral malleolus and terminates in the extensor digitorum brevis (EDB) on the dorsum of the foot. During peroneal motor NCS recording from the EDB, the peroneal CMAP amplitude is larger-stimulating proximally than distally because the anomalous fibers are not present at the ankle. Stimulation behind the lateral malleolus confirms this anomaly, which yields a small CMAP that approximately equals the difference between the CMAP amplitudes evoked with distal and proximal peroneal nerve stimulations. Complete innervation of the EDB by the accessory deep peroneal nerve is rare but should be suspected if there is preservation of function in the EDB muscle (i.e., extension of lateral toes) in a patient with severe deep peroneal neuropathy (Kayal and Katirji, 2009).
Temporal Dispersion and Phase Cancellation
The CMAP, evoked by supramaximal stimulation, represents the summation of all individual MUAPs directed to the muscle through the stimulated nerve. Typically, as the stimulus site moves proximally, the CMAP slightly drops in amplitude and area and increases in duration. The cause is temporal dispersion in which the velocity of impulses in slow-conducting fibers lags increasingly behind those of fast-conducting fibers as conduction distance increases. With dispersion, a slight positive and negative phase overlap, and cancellation of MUAP waveforms is seen (Fig. 32B.5). The result of temporal dispersion and phase cancellation is a reduction of CMAP amplitude and area and prolongation of its duration.
Physiological temporal dispersion affects the SNAP more than the CMAP (Fig. 32B.6). This difference relates to two factors. The first relates to the disparity between sensory fiber and motor fiber conduction velocities. The range of conduction velocities between the fastest and the slowest individual human myelinated sensory axons is almost twice that for the motor axons (25 m/sec and 12 m/sec, respectively). The second factor is the difference in duration of individual unit discharges between nerve and muscle. With short-duration biphasic sensory spikes, a slight latency difference could line up the positive peaks of the fast fibers with the negative peaks of the slow fibers and cancel both (Fig. 32B.7). In longer-duration MUAPs, the same latency shift would only partially superimpose peaks of opposite polarity, and phase cancellation would be less of a factor.
Electrodiagnosis by Nerve Conduction Studies
Focal Nerve Lesions
The classification of peripheral nerve lesions follows. In neurapraxia (first-degree injury), distortion of myelin occurs near the nodes of Ranvier, producing segmental conduction block without wallerian degeneration. In axonotmesis (second-degree injury), the axon is interrupted, but all the supporting nerve structures remain intact. In neurotmesis, the nerve injury is severe, resulting in complete disruption of the nerve with all the supporting structures (see Chapter 52D). Often, the neurotmesis category is divisible as follows: third-degree injury, with disruption of the endoneurium and with intact perineurium and epineurium; fourth-degree injury, with disruption of all neural elements except the epineurium; and fifth-degree nerve injury, with complete nerve transection resulting in complete discontinuity of the nerve. Electrodiagnostic studies alone cannot accurately distinguish among the five degrees of nerve injuries, but they can separate the first (neurapraxia) from the other types (Wilbourn, 2002).
Demyelinative Mononeuropathy
When focal injury to myelin occurs, conduction along the affected nerve fibers may alter. This may result in conduction slowing or block along the nerve fibers. The cause of conduction block is interruption of action potential transmission across the nerve lesion; it is the electrophysiological correlate of neurapraxia and usually results from loss of more than one myelin segment (segmental or internodal demyelination). Bracketing two stimulation points, one distal and one proximal to the site of injury, best localizes a nerve lesion with conduction block. With such lesions, stimulation distal to the lesion elicits a normal CMAP, whereas proximal stimulation evokes a response with reduced amplitude or fails to evoke any response, defined as partial or complete conduction block, respectively (Fig. 32B.8, A). Several limitations exist to the diagnosis of demyelinative conduction block:
1. Phase cancellation between peaks of opposite polarity may reduce CMAP size because of abnormally increased temporal dispersion. Such excessive desynchronization often develops in acquired demyelinative neuropathies. If the distal and the proximal responses have dissimilar waveforms, the discrepancy in amplitude or area between the two may be the result of phase cancellation rather than conduction block. Therefore, for a diagnosis of partial conduction block, findings should include a significantly lower CMAP amplitude and smaller CMAP area with stimulation proximal to the injury site than with the CMAP distal to it, and without any significant prolongation of CMAP duration. More than 50% decay of both the CMAP amplitude and area across the lesion usually is the criterion for definite conduction block.
2. Distal demyelinating lesions causing conduction block of the nerve segment between the most distal stimulating point and the recording site manifest as unelicitable or low CMAP amplitudes at both distal and proximal stimulation sites. This finding mimics the NCS seen with axonal degeneration.
3. The prominent temporal dispersion normally seen in evaluating SNAPs precludes the use of these potentials to diagnose conduction block.
4. Conduction block also may follow axonal loss before the completion of wallerian degeneration.
Focal slowing of conduction usually is the result of widening of the nodes of Ranvier (paranodal demyelination). Slowing, often synchronized, affects all large myelinated fibers equally. This results in prolongation of distal latency if the focal lesion is distal (see Fig. 32B.8, B,a), or slowing in conduction velocity if the focal lesion is proximal (see Fig. 32B.8, B,b). CMAP amplitude, duration, and area, however, are normal and do not change when the nerve is stimulated proximal to the lesion. Desynchronized slowing (differential slowing) occurs when conduction velocity reduces at the lesion site along a variable number of the medium or small nerve fibers (average- or slower-conducting axons). Here, the CMAP disperses with prolonged duration on stimulations proximal to the lesion. The speed of conduction along the injury site (latency or conduction velocity) is normal because of sparing of at least some of the fastest-conducting axons (see Fig. 32B.8, C). When synchronized and desynchronized slowing coexists, slowing of distal latency or conduction velocity accompanies the dispersed CMAP with prolonged duration.
Axon-Loss Mononeuropathy
Soon after axonal transection (i.e., for the first 48 hours), the distal axon remains excitable. Therefore, stimulation distal to the lesion elicits a normal CMAP, whereas proximal stimulation elicits a response with reduced amplitude, producing a conduction block pattern (see Fig. 32B.8, D, middle panel). This pattern is axonal noncontinuity, early axon loss, or axon-discontinuity conduction block. Soon, however, the distal axons undergo wallerian degeneration, and the distal CMAP decreases to equal the proximal CMAP (see Fig. 32B.8, D, lower panel). With wallerian degeneration, the distal CMAP decreases in amplitude starting 1 or 2 days after nerve injury and reaches its nadir in 5 to 6 days. In contrast, the distal SNAP lags slightly behind and reaches its nadir in 10 or 11 days (Fig. 32B.9). The difference between the decline of the SNAP and CMAP amplitudes after axon loss probably relates to neuromuscular transmission failure, which affects only the CMAP amplitude. Supporting this hypothesis is the fact that MNAPs recorded directly from nerve trunks follow the time course of SNAPs.
< div class='tao-gold-member'>
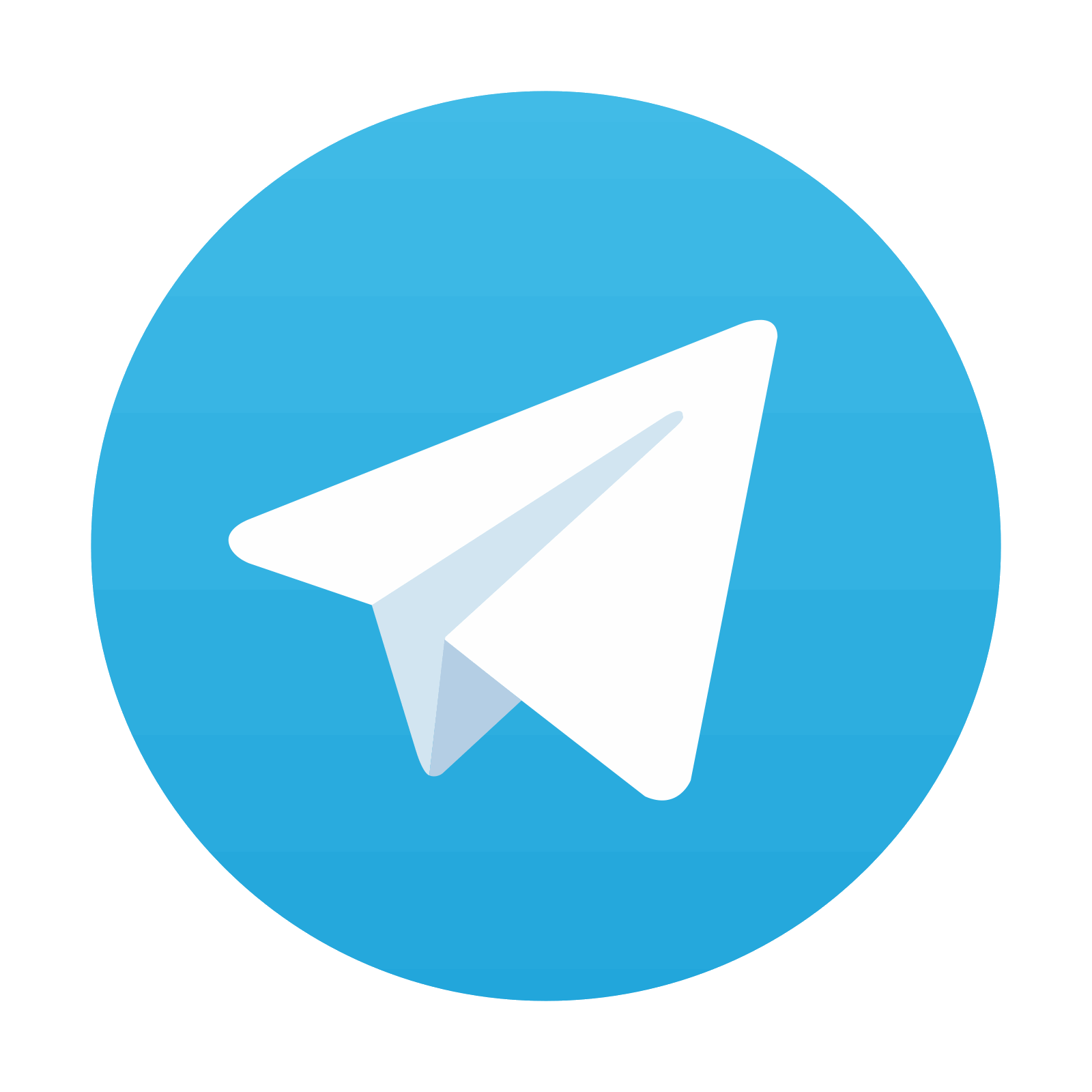
Stay updated, free articles. Join our Telegram channel
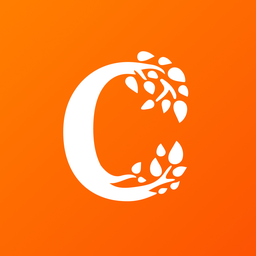
Full access? Get Clinical Tree
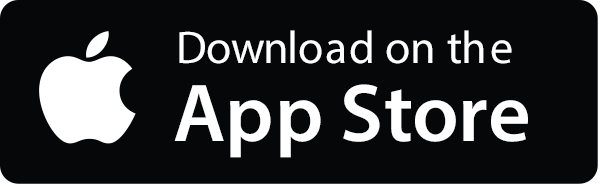
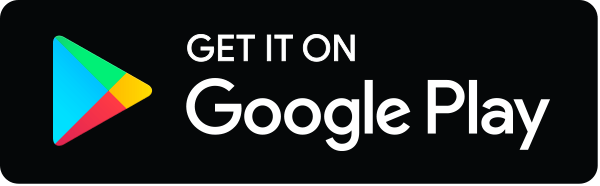