Abstract
Dystonia is etiologically classified as primary versus secondary based on the absence or presence, respectively, of an identifiable underlying cause. Deep brain stimulation (DBS) at the internal globus pallidus is currently the treatment of choice for medically refractory primary dystonia. Multiple open-label and blinded studies, along with three placebo-controlled trials, demonstrate that pallidal DBS is a highly effective, durable, and well-tolerated treatment modality in patients with primary dystonia. Younger patients with shorter disease duration and without joint contractures and those who have the DYT1 positive gene mutation may fare best of all. When prolonged dystonia has resulted in fixed contractures, additional orthopedic surgery may be required to maximize functional gains, though the results in such patients may never equal that achieved in patients without fixed deformities.
Patients with secondary dystonia respond more modestly and inconsistently than do primary dystonia patients, reflecting the physiologic and anatomic heterogeneity of this population. Among these, patients with tardive dystonia, pantothenate kinase-associated neurodegeneration, and dystonia associated with brain lesions but with preserved basal ganglia anatomy may respond well to DBS therapy. Conversely, patients with obvious structural abnormalities and most metabolic disorders appear to be poor DBS candidates.
Keywords
Deep brain stimulation, Dystonia, Stereotactic Surgery, Surgical therapy
Outline
Introduction 931
Diagnosis and Classification of Dystonia 932
Medical Therapy for Dystonia 932
Surgical Therapy for Dystonia 932
The DBS Procedure 933
Patient Selection 933
Surgical Procedure 933
Stereotactic Technique 934
Anatomical Targeting 934
Microelectrode Recording 935
DBS Surgery in Children 936
Macroelectrode Stimulation 936
Implantation of the Pulse Generator 936
Programming the Device 937
Clinical Results 937
Indicators of DBS Response 938
Stimulation Frequency 939
Longevity of Response 939
Pallidal DBS in Cervical Dystonia 939
Pallidal DBS for Primary Cranial–Cervical Dystonia (Meige Syndrome) 939
Pallidal DBS for Secondary Dystonia 939
Complications of DBS Therapy 940
Alternative Targets for Deep Brain Stimulation in Dystonia 940
Conclusions 941
Acknowledgment [CR]
References 941
Acknowledgment
The senior author wishes to thank Donald Weisz, PhD for his assistance with the production of Fig. 76.3 , for his friendship, and for his passionate commitment to patient care.
Introduction
Dystonia is a neurological disorder characterized by sustained or intermittent muscle contractions causing twisting, repetitive movements that result in abnormal, often painful, postures ( ). Different muscle groups may be involved to a variable extent and severity. Dystonia is not one disease; rather, it is a neurological manifestation of many pathological conditions, most of which are poorly characterized. The prevalence estimates for primary dystonia in the general population range from 2 to 50 cases/million for early-onset dystonia and from 30 to 7320 cases/million for late-onset dystonia ( ). However, prevalence rates are significantly higher in some ethnic groups ( ).
Due to the limitations of medical therapies for dystonia, various surgical interventions targeting both the peripheral and central nervous systems have been attempted, mostly with mixed results ( ). However, following initial case reports published in 1999, deep brain stimulation (DBS), particularly at the internal globus pallidus (GPi), has emerged as the surgical treatment of choice for medically refractory cervical, segmental, and generalized dystonia ( ).
Diagnosis and Classification of Dystonia
Dystonia may be classified in several ways, with the most recent consensus statement emphasizing two distinct axes: clinical features and etiology ( ). Several features describe the clinical characteristics of a given patient: the anatomical distribution of the abnormal movements; the age at symptom onset (early versus late); the temporal pattern of symptoms; and the coexistence of other movement disorders or neurological manifestations. Focal dystonias (e.g., writer’s cramp, spasmodic torticollis) are limited to a single body region; segmental dystonia affects more than one contiguous body part (e.g., cranio–cervical dystonia); multifocal dystonia involves noncontiguous body regions; hemidystonia denotes multiple noncontiguous body regions restricted to one side of the body; and widespread involvement of the axial and limb musculature characterizes generalized dystonia. Patients with early symptom onset (i.e., age <26) are more likely to have a heritable form of dystonia and to suffer generalized symptoms ( ). The temporal course can be static or progressive, with superimposed persistent, action-specific, diurnal, and paroxysmal patterns of manifestation. Dystonia may be combined with other movement disorders (e.g., myoclonus, Parkinsonism) or neurologic features (e.g., cognitive, psychiatric).
Dystonia is etiologically classified as primary versus secondary based on the absence or presence, respectively, of an identifiable underlying cause. Primary or idiopathic dystonia implies no identified structural brain abnormality or specific toxic, metabolic, traumatic, or infectious etiology. The heritable forms of dystonia are traditionally included in this group; however, they have been separated into their own category in the newest classification system ( ). A constantly growing list of primarily single-gene mutations are associated with primary dystonia, with dystonia being the sole or most prominent symptom ( ). The most common of these results from a GAG deletion of the gene encoding the protein torsin A ( ). This mutation, referred to as DYT1, is associated with a form of childhood-onset dystonia formerly known as dystonia musculorum deformans or Oppenheim’s disease. DYT1-associated dystonia is inherited in an autosomal dominant pattern but with a penetrance of just 30%–40%, suggesting that additional genetic and/or environmental factors contribute to the expression of the dystonic phenotype ( ).
When a structural brain abnormality or specific underlying etiology is identified, a dystonia is classified as secondary or symptomatic. Secondary dystonia is more prevalent than primary dystonia and may arise from a variety of causes, including static encephalopathy, stroke, traumatic brain injury, or any number of toxic, metabolic, or infectious disorders. Often included here is a large group of genetic syndromes that, unlike their mainly monogenic DYT counterparts, often express dystonia as a component of a complex phenotype ( ). Consequently, this is a heterogeneous patient population with varied pathophysiologies and responses to treatment ( ).
Medical Therapy for Dystonia
In most cases medical therapy for dystonia is limited to symptom control and is variably effective ( ). Physical therapy and orthotic devices can sometimes help maintain range of motion and prevent contractures. Anticholinergic medications (e.g., trihexyphenidyl) are the mainstay of medical therapy for generalized dystonias but often yield only modest improvements and, at the high doses employed for dystonia, may cause significant side-effects such as drowsiness, blurred vision, and poor memory. Additional medications for dystonia include baclofen, benzodiazepines, zolpidem, and tetrabenazine. A minority of patients with symptomatic generalized dystonia will benefit from specific therapy targeted at the underlying disorder. Children and adolescents with “clinically pure” dystonia of unknown etiology should be evaluated for Wilson’s disease and undergo a trial of levodopa therapy, as a small subpopulation with dopa-responsive dystonia will experience a profound and sustained response to this medication ( ).
Targeted injections of botulinum toxin (botox) can alleviate focal dystonias, but this intervention is impractical in patients with generalized symptoms ( ). Some patients will not respond to botox initially, and up to 10% may develop resistance over time through the production of blocking antibodies ( ).
Surgical Therapy for Dystonia
Surgical intervention for dystonia should generally be considered when a patient’s symptoms are disabling and the response to medical therapy is either inadequate or limited by side-effects. Historically, surgical interventions for dystonia have targeted both the peripheral and central nervous systems ( ). Peripheral denervation procedures for focal dystonias have largely been supplanted by chemical denervation with botox ( ). Chronic intrathecal baclofen infusions can alleviate dystonia, though existing case series report mixed motor and functional results ( ) with significant hardware-related complication rates. Intrathecal baclofen infusion is more commonly considered for mixed dystonia and spasticity, as most commonly observed in cerebral palsy.
Advances in stereotactic technique, the success of DBS in Parkinson’s disease (PD) and essential tremor (ET), and the observation that pallidotomy improves off-medication dystonia in PD patients ( ) renewed interest in basal ganglia interventions for dystonia in the 1990s. Stereotactic pallidotomy does improve symptoms of primary generalized dystonia (PGD) ( ), but unilateral pallidotomy may not sufficiently treat generalized symptoms and bilateral pallidotomy entails significant risk, including cognitive dysfunction, dysarthria, dysphagia, and limb weakness ( ). Consequently DBS, which is reversible and may be employed bilaterally with relative safety, has emerged as a preferable alternative.
The DBS Procedure
Consistently successful DBS surgery involves three critical steps: careful patient selection; precise lead implantation; and skillful device programming. Failure to perform any one of these three steps properly may lead to suboptimal results.
Patient Selection
As discussed in the introduction, dystonia is a complex group of disorders, most of which are not responsive to DBS. Thus it is important for all surgical candidates to be evaluated by a movement disorders neurologist before proceeding. He/she will ensure that the diagnosis of dystonia is correct, and that all reasonable medical therapies have been tried. At many centers the neurologist also programs the DBS devices after implantation, manages medication changes, and monitors patient progress.
In the United States Activa Dystonia Therapy (Medtronic, Inc, Minneapolis, MN, USA) is approved under a Humanitarian Device Exemption exclusively for the treatment of primary dystonia in patients over the age of 7 years. All other uses are considered to be “off-label.” Patients should not be offered surgery unless their symptoms are disabling and have failed to respond to standard medical therapies. A notable caveat to this may be select children with PGD. Here GPi DBS is increasingly considered a first-line treatment, with the goal of reducing loss of mobility and joint deformity prior to the development of contractures, and minimizing the impact of centrally acting medications on cognitive and social development ( ). A recent magnetic resonance imaging (MRI) scan of the brain should be obtained to rule out structural lesions. Patients with childhood-onset generalized dystonia should be tested for Wilson’s disease and the DYT1 mutation, and should receive an adequate trial of levodopa before proceeding with surgery.
Surgical Procedure
The implanted device has four primary components ( Fig. 76.1 ) that are implanted in two phases, either as a single or a staged operation. During the first phase, the stimulating lead(s) is implanted into the GPi stereotactically and secured by means of an anchoring system that also covers the burr hole. The remaining two components, the extension cable(s) and pulse generator(s), are implanted during the second phase of the procedure, which may be performed at the same setting or shortly (days to weeks) thereafter. It is acceptable to implant DBS leads bilaterally during the same procedure. Dystonia patients are typically much younger than patients with PD and ET, and in our experience tolerate the bilateral frontal lobe penetrations without difficulty.

Stereotactic Technique
Stereotactic headframes remain the primary means for performing DBS lead implants, but “frameless” technologies are being used with greater frequency ( ). These systems employ either a custom-built or adjustable targeting platform in concert with skull-mounted fiducials or an MRI-compatible implantation platform that allows real-time MRI guidance of the lead and target during implantation ( ). Good outcomes are reported using each of these methods, with accuracies rivaling that achieved with conventional headframes. No reports directly compare these techniques to suggest the superiority of one over another ( ). Potential advantages of frameless systems include enhanced patient comfort, workflow efficiency, and anatomical accuracy. On the other hand, stereotactic frames may provide greater head stability during surgery, compatibility with microelectrode recording platforms, entry point and trajectory flexibility, and lower procedure cost, and have the greatest published experience ( ).
Anatomical Targeting
As with stereotactic targeting technologies, a variety of imaging techniques may be used to perform DBS implants. The senior author employs axial and coronal fast-spin echo/inversion recovery (FSE/IR) MRI because the images are acquired rapidly (∼6 min/scan) and provide superior resolution of the commissures and deep nuclei ( Fig. 76.2 ; scanning parameters in Table 76.1 ). These images alone are sufficient for performing DBS implants with microelectrode guidance, but additional image sets such as gadolinium-enhanced three-dimensionally acquired T1-weighted MRI (e.g., spoiled gradient recalled acquisition (SPGR)), and/or stereotactic computerized tomography may also be employed. In our experience, both the volume error of fiducial registration and targeting accuracy are significantly improved when SPGR MRI is used for fiducial registration and FSE/IR for target selection ( ). Moreover, contrast-enhanced SPGR demonstrates the cortical veins so they may be avoided when selecting an entry point. Computerized tomography (CT) provides the most geometrically accurate images for fiducial registration and can be acquired rapidly on the day of surgery.

Excitation time (Te) | 120 ms |
Relaxation time (Tr) | 10,000 ms |
Inversion time (Ti) | 2200 ms |
Bandwidth | 20 83 |
Field of view | 24 |
Slice thickness | 3 mm |
Slice spacing | 0 mm |
Frequency | 192 Hz |
Phase | 160 |
Number of excitations | 1 |
Frequency direction | Anteroposterior |
Autocontrol frequency | Water |
Flow compensation direction | Slice direction |
The images are transferred to an independent workstation equipped with advanced stereotactic targeting software. These software packages provide at least five advantages: the target coordinates are calculated automatically, eliminating human math errors; a variety of image sets (e.g., CT and MRI) may be merged, allowing one to exploit the advantages of different types of imaging; the entire trajectory may be visualized in all three anatomical planes and orthogonal to the trajectory (so-called “probe’s-eye view”), allowing one to plan safer approaches to the target; the image data sets are reformatted orthogonally to the intercommissural plane, controlling for variations in headframe placement; and digitized versions of stereotactic atlases may be overlaid and digitally “fit” to the patient’s anatomy, helping to identify the desired target. A significant drawback to these systems is that they assume the patient’s brain to be symmetrical, which is not always the case. Congenital anomalies such as plagiocephaly can shift target structures, and it is the surgeon’s responsibility to account for these shifts during the targeting process.
We target the internal pallidal site first described by Leksell, which lies 19–22 mm lateral, 2–3 mm anterior, and 4–5 mm inferior to the midcommissural point ( ). The target should be visualized on both axial and coronal images, and should lie 2–3 mm superior and lateral to the optic tract ( Fig. 76.2B ). Our preferred trajectory is 60–65 degrees anterior and superior the intercommissural plane and 0–10 degrees lateral to the vertical axis. This trajectory allows one to avoid the ipsilateral lateral ventricle and still employ nearly parasagittal trajectories, which facilitate the process of mapping the intraoperative microelectrode recording data to human stereotactic atlases (see below).
Microelectrode Recording
The need for intraoperative neurophysiological confirmation of proper targeting remains controversial, as there are no published reports directly comparing the relative benefits and adversities of neurophysiologically guided versus nonneurophysiologically guided surgery for dystonia ( ). Acceptable outcomes have been obtained with both approaches. A detailed description of neurophysiological recording and stimulation techniques, and discussion of their potential pros and cons in GPi DBS surgery, is beyond the scope of this chapter. An overview of our use of microelectrode recording (MER) and macrostimulation is given below. The interested reader is encouraged to review the finer details of our MER technique ( ) and neurophysiological monitoring ( ) elsewhere.
The senior author employs MER to refine his anatomical targeting if necessary. In his experience, MER provides important real-time information that other localization techniques simply do not. First, MER delineates the borders and expanses of the globus pallidus pars externa (GPe) and GPi along a given trajectory with a spatial resolution of ∼100 μ. This data may be mapped on to scaled sagittal sections of human stereotactic atlases to determine the anatomic position of the recording trajectory. Acceptable trajectories for implantation include a 3–4 mm span of the GPe and at least 6 mm of the GPi. Such a trajectory must pass through the heart of the GPi and will allow three to four contacts to be positioned comfortably within the nucleus, depending on the lead employed ( Fig. 76.3 ). Second, the detection of kinesthetic cells confirms that the trajectory traverses the sensorimotor subregion of the GPi, the physiologically defined target for the procedure. Third, delineating the inferior border of the GPi refines the depth of implantation. And fourth, identifying the optic tract 2–3 mm inferior to the GPi exit point confirms that the trajectory exits the nucleus inferiorly, not posteriorly into the internal capsule. Identification of the optic tract provides an additional level of confidence that the lead will be well positioned, but this should not be viewed as an absolute requirement for implantation, as the optic tract may not be identified in many cases.

Anticholinergics and benzodiazepines may be withheld on the morning of surgery to facilitate recordings, which can be affected by these medications. Baclofen should not be withheld, however, as in the senior author’s experience this may precipitate a dystonic crisis.
DBS Surgery in Children
The first phase of the DBS procedure is conventionally performed with the patient fully awake, but this may be challenging for young children or patients with severely contorted postures. If maturity, painful muscular spasms, or abnormal postures make awake surgery arduous, DBS lead implants may be performed under conscious sedation or even full general anesthesia. It is the senior author’s experience, however, that MER-guided DBS may be performed even in children so long as one has a dedicated neuroanesthesiologist who is willing to manage the level of consciousness carefully throughout the procedure so that the patient is comfortable but awake enough to obtain useful recordings ( ).
Macroelectrode Stimulation
The DBS lead is inserted along the desired trajectory, leaving the deepest contact (contact 0) at the physiologically defined inferior border of the GPi. In the past, C-arm fluoroscopy was employed to confirm that the lead had traveled to the desired point relative to the frame ( Fig. 76.4 ), but since 2016 the senior author has adopted intraoperative CT scanning to document lead position before leaving the operating room. Before it is secured, the acute effects of stimulation via the lead are tested. Testing is performed in bipolar mode employing the parameters of pulse width 90 μs, frequency 130 Hz, and amplitude 0–4 V. Stimulation amplitudes greater than 5 V are not used, as we have never required amplitudes this great for therapy. The initial test is performed with the deepest pair of contacts (i.e., 0−, 1+), as these are closest to the internal capsule and therefore most likely to generate adverse effects (AEs). If no AEs are observed, testing continues in a ventral to dorsal sequence. Unlike PD, dystonia requires days to weeks of stimulation therapy before improvements are apparent, thus a lack of improvement in response to intraoperative stimulation should not be viewed as an indicator of poor lead placement. Rather, one must have faith that if the microelectrode recording data is consistent with good placement and there are no AEs observed with up to 4 V of stimulation, the lead is well positioned.

Sustained time- and voltage-locked contractions of the contralateral hemibody and/or face indicate that stimulation is activating the fibers of the internal capsule, in which case the lead is placed too medially and/or posteriorly. The induction of phosphenes in the contralateral visual field suggests that stimulation is activating the optic tract and the lead is too deep. Stimulation within the sensorimotor GPi may induce transient paresthesiae; however, sustained paresthesiae at low stimulation amplitudes indicate that the lead is positioned very posteriorly and is activating thalamo–cortical projections in the posterior limb of the internal capsule. If any of these AEs occur, the lead should be repositioned accordingly.
The lead is secured at the skull employing a “cap” that also covers the burr hole. Either serial fluoroscopy or intraoperative CT may be used to confirm that the lead was not displaced from its desired position during fixation. The remaining length of the lead is encircled around the burr hole cap and left in the subgaleal space. The incision is irrigated with antibiotic saline and closed in a standard fashion. After removing the stereotactic frame, the patient is transported to radiology where postoperative MRI is performed to confirm that the leads are well positioned and there has been no hemorrhage ( Fig. 76.5 ). Patients are observed overnight in the neurosurgical intensive care unit and discharged the following day.
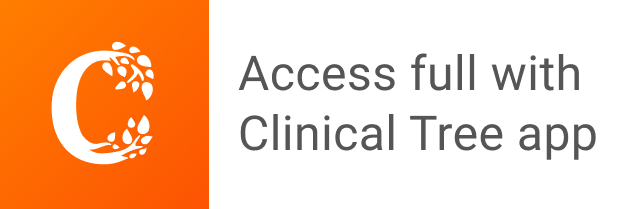