Abstract
This chapter focuses on neuromodulation systems that leverage feedback control to adjust stimulation properties automatically. Most current neuromodulation systems provide open-loop stimulation, wherein stimulation parameters are static and unaffected by changes in symptoms or disease. In contrast, closed-loop systems adapt therapy in response to physiological changes and may provide more effective and efficient therapy. Closed-loop systems have already begun to appear in clinical practice. The chapter introduces the scientific background and technology landscape for three therapy areas that are using closed-loop control: spinal cord stimulation, vagal nerve stimulation, and deep brain stimulation. A review of general design strategies and practical considerations for the development of neuromodulation systems that leverage principles of feedback control to deliver therapy is presented.
Keywords
Chronic pain, Closed loop, Deep brain stimulation, Epilepsy, Movement disorder, Nerve stimulation, Neural engineering, Spinal cord stimulation
Outline
Overview 305
Spinal Cord Stimulation for Treatment of Chronic Pain 306
Cortical and Vagus Nerve Stimulation for Treatment of Epilepsy 307
Deep Brain Stimulation for Treatment of Movement Disorders 308
Design Strategies for Future Neuromodulation Devices for Feedback Control 311
Modular and Configurable Devices 311
Flexibility Through Firmware Upgrades 311
Algorithm Prototyping 312
Application–Programming Interfaces 312
Cloud Connectivity and Data Analytics 312
References 312
Overview
Neural stimulation is an established therapy for the treatment of chronic pain ( ), movement disorders ( ), epilepsy ( ), and urinary and bowel control ( ), and is now being investigated for numerous other psychological and neurological disorders. For many neuromodulation systems therapy parameter determination is limited to physician programming, resulting in parameters that are static between clinic visits, potentially for months at a time. Programming adjustments can lag patient need considerably, because fluctuations in disease symptoms or the user’s body can change within weeks, days, or hours. To allow more flexible therapy, most systems give patients the ability to change stimulation parameters manually, with a choice of preset programs within preset ranges. However, manual adjustment does not ensure optimal therapy use and places the burden on the patient. Devices that automatically change in response to patient need ( Fig. 23.1 ) may help to provide consistent therapy, reduce the side-effects of overtreatment or undertreatment, and improve battery longevity by providing therapy only when needed. This sort of automaticity may also lower the burden associated with manual adjustments.

Systems that adapt therapy in response to physiological changes have already begun to appear in clinical practice ( ). This review focuses on the current status of three neuromodulation therapies that leverage closed-loop control: spinal cord stimulation (SCS) for treatment of pain; cortical and vagus nerve stimulation for treatment of epilepsy; and deep brain stimulation (DBS) for treatment of movement disorders. Each section reviews the history and scientific background of the therapy, and then examines the current state of closed-loop control. Finally, looking forward to the needs of new systems, a set of strategies that can be used in developing new systems is presented.
Spinal Cord Stimulation for Treatment of Chronic Pain
History and Scientific Evidence
First approved by the United States Food and Drug Administration (FDA) in 1989, SCS has become a common intervention for patients with chronic pain in their back or limbs and represents nearly 70% of all neuromodulation treatments ( ). Despite the significant therapeutic benefit of the open-loop therapy, patients use their remote programmers to adjust for changes in pain intensity or body posture. Changes in body position are known to affect the perceived stimulation effect ( ). At the same stimulation settings, the paresthesia is usually perceived more intensely in the supine position than in an upright position. This position dependency is thought to be due to changes in the thickness of the cerebrospinal fluid layer around the spinal cord rather than to dislocation of the electrode or changes in electrode impedance ( ). Automatic adjustment of stimulation intensity and other parameters such as electrode configuration to account for spinal cord movement would help maintain consistent therapy and lower the burden associated with frequent use of the remote programmer.
At least two feedback signals have been identified for closed-loop control of stimulation adjustments related to spinal cord movement. For adapting stimulation based on body posture, motion sensors such as accelerometers can be used to provide a straightforward feedback signal for the control policy ( ). Feedback based on accelerometry, however, only addresses therapy needs when defined patient positions change; it does not address spinal cord movement in a static position or rotational spine or body movement. Electrophysiological biomarkers have been investigated as a mechanism to monitor these aspects of spinal cord stimulation objectively. Recordings of the evoked compound action potential (ECAP) from epidural electrodes in humans have shown some potential as an indicator of pain and anatomy ( ). A correlation between ECAP magnitude and thresholds for stimulation was demonstrated, showing that ECAPs may provide appropriate feedback on therapy parameters. Additionally, the magnitude of the ECAP was correlated with the degree of coverage of the painful area. ECAP localization may help in finding the best position for electrode implantation. This feedback during the lead placement procedure has the potential to provide clinical data in real time to enable optimal and objective placement of the electrode with respect to the spinal cord, without having to rely solely on patient cues.
Technology Landscape
Medtronic RestoreSensor
The RestoreSensor system uses a three-axis accelerometer to sense patient body position and automatically adjust the stimulation settings ( Fig. 23.2 ). An initial calibration is required, during which the patient is positioned in multiple orientations (e.g., standing, lying prone, lying supine) and appropriate stimulation settings are identified for each position. As the position changes, preferred stimulation settings are found by adjusting the amplitudes of multiple programs. By using multiple programs, the parameters of amplitude, pulse width, rate, and electrodes can be tailored to a patient’s therapy needs. In a clinical study involving 79 patients implanted with the RestoreSensor system, 86.5% of patients achieved the primary objective of improved pain relief with no loss of convenience or improved convenience with no loss of pain relief using automatic position-adaptive stimulation compared to using conventional manual programming adjustment alone ( ). Additionally, position-adaptive stimulation demonstrated a statistically significant 41% reduction in the daily average number of programming button presses for amplitude adjustment compared with manual programming. The adverse event profiles in both treatment groups were similar, and no unanticipated adverse device effects were reported. The study demonstrated that automatic position-adaptive stimulation is safe and effective in providing benefits in terms of patient-reported improved pain relief and convenience compared with using manual programming adjustment alone.

Saluda Evoke
Saluda Medical is currently developing a closed-loop system (Evoke) to adjust stimulation automatically for pain relief using the ECAP as a feedback signal. In acute evaluations of SCS with feedback control, seven of eight subjects evaluated had fewer side-effects and smaller variation in response when compared to conventional fixed-current stimulation ( ). A larger-scale chronic study is currently under way ( ).
Cortical and Vagus Nerve Stimulation for Treatment of Epilepsy
History and Scientific Evidence
Epilepsy is characterized by recurrent seizures, and is the third most common neurological disorder in the world ( ). Penfield and Jasper reported a series of acute experiments in 1954, during which they observed that in some cases electrical stimulation of the cortex resulted in a flattening of the local electrocorticogram (both normal rhythms and spontaneous epileptiform discharges) ( ). Since then neural stimulation has become a treatment option for the 15%–40% of patients with forms of epilepsy that are resistant to drugs and surgery ( ). The FDA has approved several implantable neurostimulation therapies for epilepsy, including an open-loop stimulator that targets the vagus nerve ( ) and a responsive system that targets cortical seizure foci ( ). The historical development of these systems is outlined below.
The earliest prototypes of closed-loop epilepsy therapies were large external systems that were tested in patients undergoing intracranial monitoring ( ). These systems interfaced with implanted electrodes for monitoring brain activity and included algorithms for detecting seizure onset. Despite the small number of subjects in these feasibility trials, stimulation in response to epileptiform activity reduced the number of clinical seizures and suppressed electrographic seizures. Vagus nerve stimulation also showed promise for a feedback-based approach. In initial clinical trials a feature was provided for user-initiated stimulation where subjects or their caregivers could pass a magnet across the implanted device in the chest at the time of the perceived seizure onset. A review of these studies showed a weighted average patient- or caregiver-reported benefit for 45% of patients, with seizure cessation in 28% ( ). An automated system based on seizure detection would reduce the burden associated with manual application of the magnet. However, due to the anatomical location of the implant for vagal stimulation, recording brain activity directly for seizure detection is not practical. Cardiac-based seizure detection is a more feasible option from a design and implementation perspective, and relies on changes in the heart rate for detection. The most frequently reported type of seizure-related cardiac change is ictal tachycardia, or an increase in heart rate with seizure, which occurs in the majority of seizures ( ). A closed-loop system would in principle stimulate only when triggered by an indicator of seizure activity. Thus the nervous system would receive less stimulation than it would with constant-stimulation open-loop strategies. This reduction in stimulation dose not only helps the battery last longer, but also decreases the potential risk of side-effects from long-term continuous stimulation ( ).
Technology Landscape
Two responsive systems are now commercially released for the treatment of refractory epilepsy: the AspireSR vagus nerve stimulator from Cyberonics responds to variations in the cardiac signal, while the Responsive Neuromodulation System (RNS) from Neuropace uses neurological signatures from field potentials recorded in the brain to initiate stimulation. Vagal nerve stimulation (VNS) activates the vagus nerve, which is believed to modulate plasticity within the central nervous system, while the RNS attempts to stimulate proximal to the seizure focus. In both cases clinical evidence suggests that feedback-based stimulation can reduce seizure frequency, is well tolerated, and is acceptably safe.
Cyberonics AspireSR
The AspireSR System leverages the predicate VNS system design to stimulate the vagus nerve, and includes onboard heartbeat-sensing technology to allow detection and monitoring of changes in heart rate ( ). The detection algorithm establishes a baseline (background) heart rate over a period of approximately 5 min as well as a near-term (foreground) heart rate for comparison. Detection (the AutoStim feature) occurs when the foreground heart rate exceeds the background heart rate by the threshold programmed by the clinician. In a clinical study of 20 patients using the AutoStim feature, no unanticipated adverse events and no new seizure types were reported ( ). Seizure severity decreased and several indicators of well-being improved over baseline at the 3-, 6-, and 12-month visits. Overall quality of life improved more than three times the minimally important clinical criteria. Median percentage change from baseline seizure frequency declined over the 3-, 6-, and 12-month visits.
Neuropace RNS
The Neuropace RNS is a cranially implanted neurostimulator that delivers stimulation to one or two seizure foci when specific electrocortigographic patterns are sensed. Three detectors that leverage distinct morphological features are provided, with configurable parameters that clinicians can use to adjust the sensitivity, specificity, and latency of detection ( ). In a long-term study of over 200 patients, seizure frequency was reduced by 44% at 1 year and 53% at 2 years, and ranged from 48% to 66% over postimplant years 3–6 ( ). Improvements in quality of life were maintained ( ), with a subset of patients also showing improvement in memory and language ( ). The most common serious device-related adverse events over the follow-up were implant site infection (9.0%) and removal of the implant (4.7%) ( ).
Deep Brain Stimulation for Treatment of Movement Disorders
History and Scientific Evidence
The FDA approved DBS for the treatment of tremor in 1997 ( ). Since then DBS has become an important treatment option for patients with Parkinson’s disease (PD), essential tremor (ET), and dystonia ( ) whose motor symptoms are not effectively managed by medication alone. While software programming guides with embedded computational models have been leveraged to streamline the programming process ( ), these tools have yet to become standard in care and do not address the temporal fluctuations that patients experience outside the clinic. Electrophysiological sensing of brain networks, however, has the potential to provide automatic adjustments across multiple scales of time.
Collectively, the scientific research completed since DBS therapy began provides robust evidence sustaining the correlation between local field potential (LFP) oscillations and the patient’s clinical state in PD ( ). Two specific electrophysiological biomarkers that have been identified are beta (13–30 Hz) and gamma (60–90) rhythms. Beta-band LFP activity has been demonstrated to be a biomarker for motor status in PD. DBS has also been shown to modulate beta synchrony ( ), suggesting that it could be used as an actuator to drive changes in motor improvement in a dynamic closed-loop fashion. In a study focusing on patients with frequent dyskinesia, dyskinesia was associated with a narrow-band gamma oscillation in the motor cortex between 60 and 90 Hz, a similar, though weaker, oscillation in subthalamic nucleus, and strong phase coherence between the two ( ). The finding of a brain rhythm reliably associated with dyskinesia has translational potential as a control signal in closed-loop DBS ( ), since stimulation-induced dyskinesia can be an important dose-limiting effect of DBS ( ). Besides disease state, brain oscillations may provide information related to behavior and volitional movement. In the case of ET, given that these patients only experience tremor during volitional movement, a feasible goal for a closed-loop neuromodulation system would be to limit DBS stimulation to just these periods of intentional movement ( Fig. 23.3 ). Desynchronization of cortical LFP in the beta band can be used to classify periods of movement versus rest ( ), and is currently being investigated as a biomarker in early feasibility human studies.
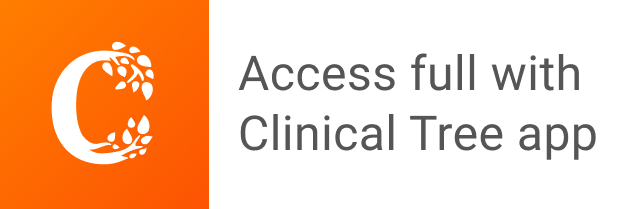