Fig. 4.1
Gastrulation and primary neurulation Embryo stages from development of Hensen’s node on day 13 to closure of anterior neuropore on day 25
4.1.2 Primary Neurulation
Further development of the neural plate begins the process of primary neurulation. This sees the plate begins to invaginate, between neural folds on each side of the midline. Cells at the top of the neural folds are referred to as neural crest cells. The neural folds then begin to fuse at several points, concomitant with the appearance of the budding somites .1
The main driving force for the shaping of the neural plate seems to be a medially directed movement of cells, with intercalation in the midline, leading to a narrowing and lengthening of the plate, a process known as convergent extension 2 (Keller et al. 2000; Copp et al. 2003), illustrated in Fig. 4.2. The result of this process is the formation of a tubular structure, below the surface of the ectoderm, by week 4 of development (Table 4.1). The cranial end of this neural tube (the anterior neuropore ) seals by the 25th day, and its location, in the mature central nervous system, is represented by the lamina terminalis . The caudal end of the neural tube (the posterior neuropore ) closes between 26 and 28 days (Norman et al. 1995) (Fig. 4.3).
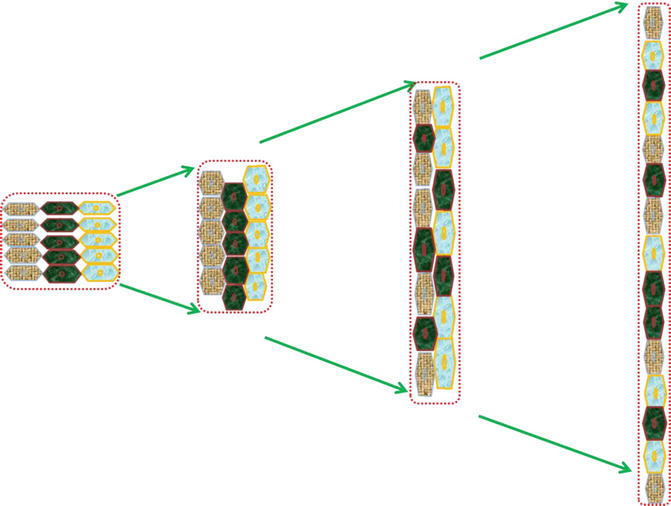
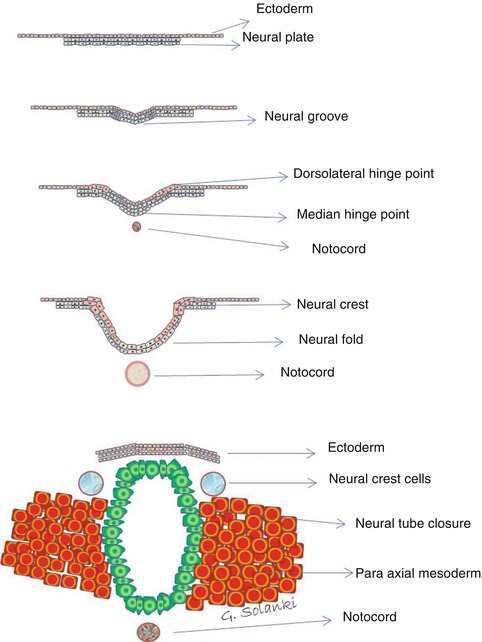
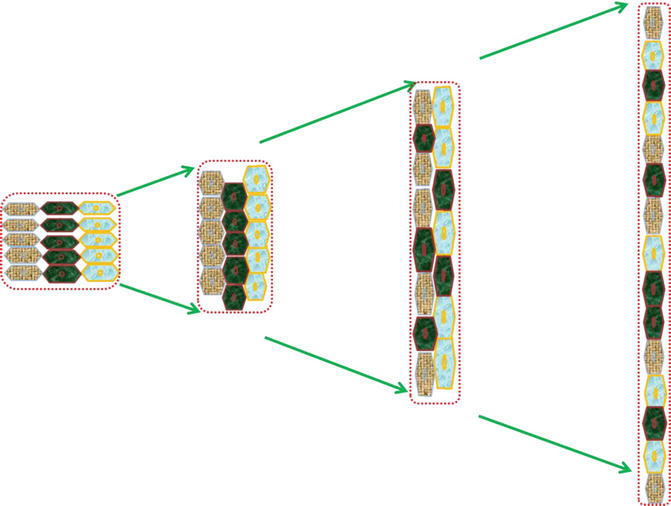
Fig. 4.2
Illustration depicting the mechanism of convergent extension . This results in gene-regulated convergence (narrowing) along one axis and extension (lengthening or elongation) in a perpendicular axis. The three cell layers first converge and intercalate causing constriction. Further morphogenesis occurs with contraction of exact cell boundaries perpendicular to growth direction and redistribution of cells into two layers along the growth axis with elongation in a sequential fashion. Finally reducing the cellular layer numbers on one axis (convergence) and adding these cells along the perpendicular elongation axis causes extension. Both gastrulation and primary neurulation cellular morphogenesis depend on effective convergent extension
Table 4.1
Stages in cranial neural tube closure
Shape of neural tube | Stage | Process |
---|---|---|
Biconvex | Inner biconvex morphology of neural folds | Expansion of the cranial mesoderm |
Transitional | Dorsolateral neural plate bending | Under sonic hedgehog homologue signalling the apices of the neural folds begin to come into apposition in the dorsal midline |
Biconcave | Midline approximation of neural folds | Contraction of subapical actin microfilaments is thought to be the process that pulls the folds together towards the midline |
Dorsal neural crest cell migration | Emigration of the neural crest occurs as the midline approximates and thins down. Timing differs between cranial and spinal ends | |
Ventral plate neuroepithelial cell deposition | Maintenance of a proliferative neuroepithelium | |
Tubular | Midline neural plate | Believed to be an apoptotic process |
Neural tube separation from dorsal epithelium | Apical apoptotic cell death is involved in epithelial remodelling following closure |
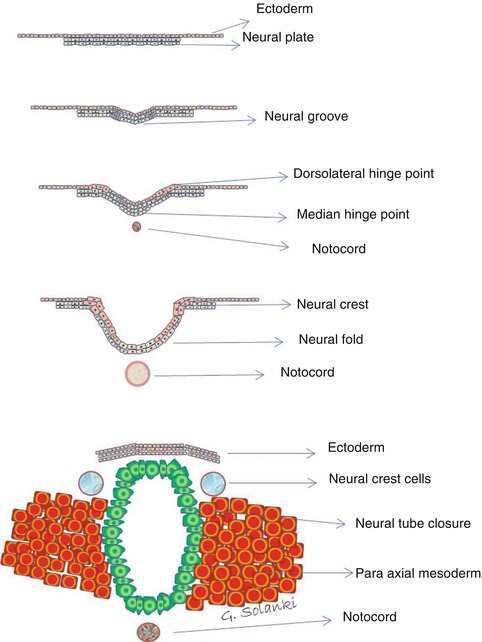
Fig. 4.3
Primary neurulation . The ectoderm germ layer by a process of columnarisation forms a thickening, the flat neural plate. The neural plate then grooves and develops a medial hinge point, folds in upon itself, developing bilateral dorsolateral hinge points and neural crests. As the neural folds are pushed upwards and towards the midline by the expanding paraxial mesoderm , the neural crest cells separate from the neuroectoderm which begins to separate from the ectoderm by a process of apoptosis. This process is achieved by neural plate switching from expressing E-cadherin to N-cadherin and N-CAM expression. This allows the approximating neural tube in the midline to recognise as the same tissue and close the neural tube
This infolding of the neural plate is usually described as starting at the craniocervical junction and proceeding both rostrally and caudally, in a ‘zipper’-like fashion. Recent evidence suggests, however, that the closure actually occurs simultaneously at multiple sites. In the mouse embryo, for example, neural tube closure initiates at three distinct locations, with an intermittent pattern of subsequent closure (Golden and Chernoff 1993). A study of neural tube defects in human embryos indicated that five closure sites exist (Van Allen et al. 1993), and other investigators have confirmed these views (Ahmad and Mahapatra 2009; Nakatsu et al. 2000), suggesting that the mode of closure in humans is different from that in other animal species. Sites identified as points of initiation include the future cervical region, the mesencephalic-rhombencephalic boundary, the anterior neuropore and the posterior neuropore. The existence of multiple simultaneous closure points help to explain why neural tube defects occur preferentially at certain sites. Examples include the lumbosacral myelomeningocoele and frontal and occipital meningo-encephalocoeles.
It is also becoming clear that neural tube closure is dependent on an apoptotic process, rather than just a proliferative growth of cells that meet in the midline. In an experimental study in chicken embryos, the apoptosis inhibitor Zvad-fmk 3 was shown to prevent cell death in the neural plate and to inhibit neural tube closure. Furthermore, the way in which brain and spinal cord components of the neural tube close differs. In the spinal region, the neural tube closes first, and outward migration of neural crest cells only begins several hours after this process is complete (Franz 1992). This contrasts with the cranial closure, where outward migration of the neural crest cells occurs before closure. Indeed, it is likely that neural crest migration is required to trigger the neural tube closure at this level.
Primary neurulation subserves the future development of the whole of the central nervous system. As a result, the vast majority of CNS anomalies, ranging from fatal deformities such as anencephaly to open neural tube defects, occur during this stage. The causes of most of these brain anomalies are still unknown (Norman et al. 1995), although advances in genetics and developmental embryology, as well as various clinical studies, looking at congenital conditions and their causes, genetic or otherwise, have found new mutations. Their effects on children and adults with brain anomalies or malfunctions are identifying an increasing number of responsible chromosomal aberrations, single gene mutations and extrinsic teratogens .
The period of time for which a deformed embryo survives is determined by the type and location of the neural tube defects. Almost all embryos with total dysraphism die by 5 weeks of gestation, and those with an opening over the rhombencephalon die by 6.5 weeks. In contrast, those with a defect at the frontal and parietal regions may survive beyond 7 weeks (Nakatsu et al. 2000). For example, even when there is severe failure of neural tube closure anteriorly, such as leading to anencephaly, the foetus may survive even to birth, although the condition is always fatal thereafter. This suggests that, in terms of survival of an embryo, normal development of the hindbrain is more important than development of the forebrain or the distal spine.
Spina bifida occurs from failure of posterior tube closure. Two varieties may arise, referred to as spina bifida aperta and spina bifida occulta. In the former, the neural elements are openly exposed with sometimes leaking CSF through thin dysplastic skin. Its most severe form leads to an open neural tube placode with cauda equina nerves lying outside of the spinal canal in a myelomeningocoele pouch. Less severe forms include dermal sinuses and meningocoele sacs. In spinal bifida occulta the anomaly includes open laminae and perhaps a neural tube lesion but covered by intact muscle and skin.
4.1.3 Molecular Control of Primary Neurulation
Differentiation of ectodermal cells into skin cells is regulated by the action of a protein, known as bone morphogenetic protein (BMP). Normally BMP4 causes ectodermal cells to differentiate into epidermis. During neural induction, however, two proteins, known as Noggin and Chordin , are produced by the notochord and its enveloping mesoderm. They diffuse locally into the overlying ectoderm and inhibit the activity of BMP4, allowing these cells to differentiate into neural cells. Thereafter closure of the dorsal neural tube is patterned in two stages, midline neural plate closure and neural tube separation from the dorsal epithelium. It is believed that these processes are brought about by a combination of programmed cell death, on the one hand, and epithelial remodelling, on the other hand, probably modulated once again by BMP4.
Development of the dorsal neural plate (the alar plate ) is controlled by its flanking ectodermal plate. Initial growth of the ventral part (the basal plate ) is organised by the notochord, which regulates much of the development of the nervous system (Jessell et al. 2000). The ventral neural tube is subsequently patterned by the protein sonic hedgehog homologue (SHH).4 Sonic hedgehog plays a key role in regulating vertebrate organ formation, including organisation of the brain and growth of digits on limbs. It also controls cell division of adult stem cells and has been implicated in the development of some cancers, such as medulloblastomas, which mostly occur in the region of the hindbrain. Sonic hedgehog can function in different ways, according to the cellular substrate upon which it acts. It also has different effects on the cells of the developing embryo, depending on its concentration. Basal (floor) plate-derived SHH subsequently signals to other cells in the neural tube and is essential for proper specification of ventral neuron progenitor domains.
SHH binds to a protein, named protein-patched homologue 1 (PTCH1). This then results in uncoupling of PTCH from a receptor named smoothened . This in turn results in activation of the Gli family of transcription factors (Gli1, Gli2 and Gli3), which are the ultimate effectors of this SHH signalling. In this context SHH acts as a morphogen, inducing cell differentiation dependent on its concentration. At low concentrations it promotes formation of ventral interneurons; at higher concentrations it induces motor neuron development, and at highest concentrations it induces floor plate differentiation. Failure of SHH-modulated differentiation results in holoprosencephaly , a condition where there is failure of midline clefting of the forebrain, with cortex crossing the midline, often associated with agenesis of the corpus callosum and a single midline thalamic mass.
4.2 Development of the Spinal Cord
As the spinal part of the neural tube develops, neuroblasts proliferate in two zones, creating the characteristic butterfly-shaped mantle of grey matter seen in cross section. The lateral walls of the tube thicken but leave a shallow, internal, longitudinal groove called the sulcus limitans , which separates the developing grey matter into a dorsal (alar) plate and a ventral (basal) plate. The sulcus limitans extends the length of the spinal cord and beyond to the mesencephalon. Cell bodies in the alar plate form the nuclei, which make up the uninterrupted dorsal column of grey matter (Fig. 4.4). These nuclei receive and relay input from somatic and visceral afferent neurons, whose fibres run in the dorsal roots of spinal nerves. In the basal plate, cells likewise form an uninterrupted column of ventral grey matter that extends the length of the cord. Axons of these efferent neurons project motor fibres to skeletal muscle and make up the ventral roots of the spinal nerves. Further proliferation and bulging of alar and basal plates results in the formation of the external longitudinally running dorsal median septum and ventral median sulcus. Concurrently the lumen of the neural tube becomes reduced to a small central canal . Addition of longitudinally running intersegmental axons, long ascending and descending axons and incoming dorsal root sensory fibres, on the outside of this grey matter, creates a marginal layer. Beginning in the 4th month, these fibres acquire myelin sheaths and form the white matter of the cord.
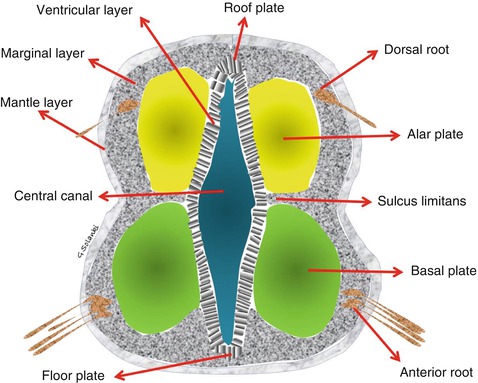
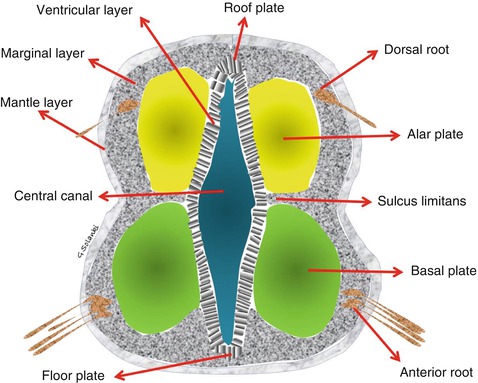
Fig. 4.4
The spinal cord Three layers (ventricular, mantle and marginal) develop from the neural tube. The ventricular layer contains undifferentiated neurons. The grey matter of the spinal cord will develop from differentiating neurons in the mantle layer and the white matter from the nerve fibres in the marginal layer
4.3 Formation of the Brain
Progressive dilatation and folding into flexures of the cranial end of the neural tube creates three distinct, primitive brain vesicles, the prosencephalon , the mesencephalon and the rhombencephalon . The mesencephalon remains undivided, to form the future cerebral peduncles and quadrigeminal plate. The alar and basal plates of the prosencephalon, on the other hand, will divide to form the telencephalon and diencephalon , respectively. The optic vesicles , which will develop into the optic nerves, retinas and irises, expand out from lateral extensions of the diencephalon. The cerebral hemispheres develop from the dorsal alar plate of the telencephalon. The basal and alar rhombencephalic plates will form the metencephalon (future pons and cerebellum) and myelencephalon (future medulla oblongata). Different parts of the future basal ganglia (nuclei basales) arise separately, the caudate nucleus and the putamen from the alar plate telencephalon and the globus pallidus, from basal plate diencephalon. The thalamus and hypothalamus also arise from basal plate diencephalon.
4.3.1 The Brainstem
Neuroblasts of the brainstem develop in a manner similar to those in the spinal cord. Alar and basal plates form sensory and motor columns of cells that supply cranial nerves, but the topographical layout of these nuclei differs in the brainstem, as compared with the cord. The mesencephalon remains undivided and consists of the basal midbrain and alar quadrigeminal plate. The pons consists of two parts, the basis pontis and the pontine tegmentum . The former is ventrally located and is phylogenetically newer. The latter is the older portion, is dorsal in position and is continuous with the medulla. The pontine tegmentum and the medulla together form the floor of the fourth ventricle. Here, the alar and basal plates are separated by a sulcus limitans , but unlike in the spinal cord, they are disposed laterally and medially, instead of dorsal and ventral. With continued development, alar and basal plates shift laterally but retain their respective functions, with the alar plates containing afferent nuclei and the basal plates forming efferent nuclei. Portions of the alar plate migrate ventrally and form the inferior olivary nucleus. Nuclei of the basis pontis migrate there from the alar plate. They receive synapses of cortically originating fibres. Medullary pyramids consist of fibres from the cerebral cortex and develop on the ventral surface near the midline
4.3.2 The Cerebellum
Caudal to the mesencephalon lies the metencephalon, which is the rostral portion of the hindbrain. It differentiates into two major structures, the cerebellum and the pons. At the rostral edge of the roof of the fourth ventricle lie the rhombic lips, which arise from the dorsolateral alar plates of the rhombencephalon. At about the fifth or sixth week, these lips start forming the cerebellar primordia . Their growth and infolding into each other causes them to fuse in the midline, creating the cerebellar plate, which covers the fourth ventricle caudal to the mesencephalon. Although the cerebellum accounts for approximately 10 % of the human brain’s volume, it contains over 50 % of the total number of neurons in the brain. The cerebellum, like the frontal lobe, is the last of the structures to develop. Chiari tonsillar descent, for example, has not been identified earlier than 10 weeks on antenatal ultrasound scans (Blaas et al. 2000). Another example of the effect of disordered development and growth on this late maturation is seen in severe prematurity,5 where cerebellar function and volume may be affected. Recent evidence shows that individuals born very preterm have significantly smaller cerebella than their term-born peers, and that this difference remains statistically significant after controlling for whole brain volume and other potentially confounding variables (Allin et al. 2001).
4.3.3 The Cranial Nerves
By the 5th week of gestation, all cranial nerves are recognisable except for the olfactory and optic nerves. The pure motor cranial nerves (III, IV, VI and XII) have no external ganglia and arise from the basal (motor) plate. Sensory nerves have conspicuous ganglia near the brain and most have motor components, except for the eighth. Apart from the third and fourth cranial nerves, which arise from the midbrain, the 5th to the 12th cranial nerves arise from the rhombencephalon (Table 4.2). Hox genes play an important role in temporospatial development of motor neurons of the trigeminal and facial nerves, as we will see later.
Table 4.2
Components of the basal and alar plates
Basal plate components | Alar plate components |
---|---|
Nucleus of cranial nerve VI | Vestibulocochlear components of cranial nerve VIII |
Motor components to muscle of branchiomeric origin of cranial nerves V and VII | Trigeminal sensory for pain and temperature, cranial nerve V |
Superior salivary nucleus of cranial nerve VII | Solitary nucleus for taste and visceral sensation of cranial nerves VII, IX and X |
Pontine nuclei in the basis pontis upon which corticofugal fibres terminate |
4.3.4 The Ventricular System
The cranial part of the neural canal (lumen of neural tube) forms the ventricular system of the brain. The shape of the ventricles is determined by the brain folding around the two primary flexures (cephalic and cervical), forming three primitive vesicles, during week 4 of gestation. These bends arise as a result of tremendous cell proliferation, occurring within the confined space of the cranial vault, causing the neural tube to buckle as the brain develops. Towards the end of week 4 and early into week 5, the primitive 3-vesicle brain divides further to become a 5-vesicle structure. Each vesicle contains its own ventricle (Table 4.3). The prosencephalon gives rise to paired lateral telencephalic vesicles , which become the cerebral hemispheres. It also forms the diencephalon, from which the optic vesicles also extend. During week 6, in the 5-vesicle stage, the pontine flexure develops. This divides the rhombencephalon into a rostral metencephalon, which will form the pons and cerebellum, and a caudal myelencephalon, which becomes the medulla. Later, the disproportionate expansion of the cerebral hemispheres alters the configuration of the lateral ventricles , which become ‘C’-shaped. These flexures also create specific narrowings within the ventricles. The foramina of Monro are located at the level of the telencephalon/diencephalon division. The cerebral aqueduct remains as a relatively simple tubular channel within the unflexed mesencephalon. During the fifth and sixth weeks, the roof of the fourth ventricle thins out in the midline to form the foramen of Magendie and, laterally, the foramen of Luschka (Melsen 1974; Koseki et al. 1993). By approximately the 7th week, a connection between the fourth ventricle and the subarachnoid space is established. The foramina of Luschka and Magendie lie at the division of the rostral metencephalon and caudal myelencephalon. More caudally, below the cervical flexure, the central canal lies within and along the spinal cord.
Table 4.3
Development of the flexures, ventricular system and foramina
Timing | Event | Flexures | Primitive vesicles | Secondary vesicles | Future ventricles |
---|---|---|---|---|---|
Early 4th week | Neural tube closure and primary neurulation start | Cephalic and Cervical | Prosencephalon | ||
Day 22 | Mesencephalon | ||||
Rhombencephalon | |||||
5th week | Primary vesicles subdivide. Future foramina of Monro will form between forebrain and midbrain | Cephalic | Prosencephalon | Telencephalic | Paired lateral ventricles |
Diencephalic | Third ventricle | ||||
Does not divide | Mesencephalon | Mesencephalic | Cerebral aqueduct | ||
Pontine flexure develops. Separates pons and cerebellum from medulla at level of future foramina of Luschka and Magendie | Pontine | Rhombencephalon | Metencephalic | Fourth ventricle | |
Myelencephalic | |||||
Cervical or cervicomedullary flexure separates medulla from the spinal cord (future foramen magnum) | Cervical | Vestigial central canal of the spinal cord |
The development of the three primary vesicles (5th week) and subsequently, at 7 weeks, the five secondary vesicles (telencephalon, diencephalon, mesencephalon, metencephalon and myelencephalon) is accomplished by the development of flexures. These flexures may also help maintain the CSF between them in a state of tension, so as to expand the ventricular system in an asymmetric way. Our ventricular system was moulded by flexural kinks into different shapes and sizes, aided by the moulding weight of the developing brain around it (Fig. 4.5). The hydrostatic tension within the ventricles acts as a vital scaffold upon which the parenchyma develops and grows. Premature unfolding of a particular flexure will impact on the CSF tension within the corresponding vesicle and almost certainly cause a degree of collapse of the dorsal structures upon it. Hypothetically, if the distal pontine flexure – which develops around the seventh week, between the pons, cerebellum and medulla oblongata – and/or the cervical flexure were to ‘unkink’, then this could result in lesser tension in the metencephalic vesicle and a smaller posterior fossa, as seen in occipital somite (skull base) development anomalies. On the other hand, if there was a continuous caudal CSF leak, as we see with leaking myelomeningocoeles, then the normal tension within the ventricular system would be reduced. The effect would be a sequential collapse of dorsal structures upon the floppy metencephalic and myelencephalic vesicle centres, causing a small posterior fossa and its caudal dislocation. An inward suction/decompression effect on the most rostral part of the ventricle could also occur, leading to flattening of the forehead. This may hypothetically explain why foetuses develop the typical lemon appearance of the forehead and the banana sign 6 on ultrasounds of myelomeningocoele and Chiari II malformation. It may also help explain the tectal beaking and neuronal migration disorders seen in association with Chiari II malformations and myelomeningocoele.
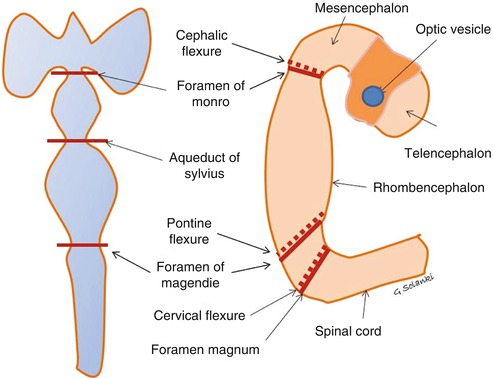
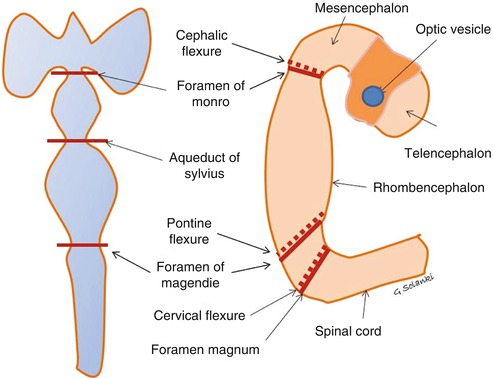
Fig. 4.5
Development of the ventricular system Neural canal develops two flexures and three vesicles. Remodelling and mantle laying of grey matter deforms the canal further into three flexures and five vesicles. The two original flexures are at the level of the foramen of Monro and foramen magnum. Above the future foramina of Monro , the prosencephalon divides into two vesicles, the mesencephalon and diencephalon . A further flexure, known as the pontine flexure, develops in the rhombencephalon, splitting it into a rostral metencephalon (the future pons and cerebellum) and a caudal myelencephalon (the future medulla oblongata). This occurs at the level of the future lateral foramina of Luschka and medial foramen of Magendie. Flexures help maintain tension within the ventricles, which may act as an internal scaffold for the mantle layer cellular proliferation
4.4 Development of Mesodermal Elements
The neural tube and its future coverings develop hand in hand. As the folding of the neural tube progresses, it becomes surrounded ventrally by the mesoderm-derived notochord, dorsolaterally by the paraxial mesoderm and neural crest cells and in the midline dorsally, by the ectoderm.
4.4.1 Somite Development
Somites are masses of mesoderm, distributed along the two sides of the neural tube, that will eventually become dermis (dermatome ), skeletal muscle (myotome ) and vertebrae (sclerotome ). During the 4th week of gestation, 42 somites are formed.. These are made up of 4 occipital somites, 8 cervical, 12 thoracic and 5 lumbar; the remainder are sacrococcygeal (Muller and O’Rahilly 1980; Gasser 1976; Arcy 1965) . Each somite then differentiates into an outer dermatome, an inner myotome and a medial sclerotome (Fig. 4.6). Because the sclerotome differentiates before the other two components, the term ‘dermomyotome ’ is sometimes used to describe the combined dermatome and myotome. Each sclerotome has three parts, a hypocentrum , a centrum and a neural arch . The first four sclerotomes go on to form the skull base and the foramen magnum. The hypocentrum forms different structures at each level (see Table 4.4). The sclerotomes are ventromedial to the neural tube and will surround the notochord and go on to form the vertebral bodies. This topography means that the skull base develops ventral to the rostral notochord (Melsen 1974).
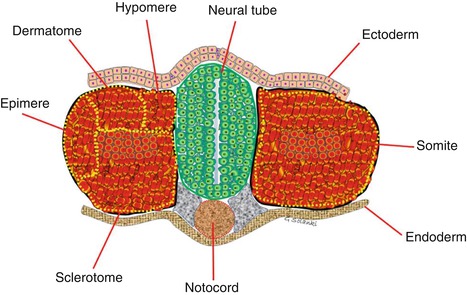
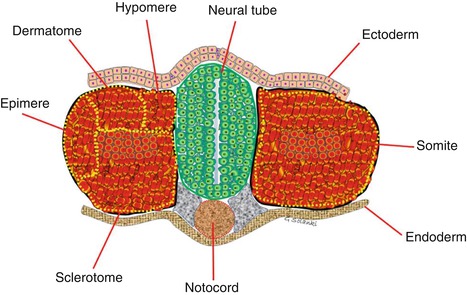
Fig. 4.6
Budding somite derived from paraxial mesoderm The sclerotome lies ventro-medially, adjacent to the neural tube. After its detachment the remaining somite is known as dermomyotome . The dermomyotome splits to form the dermatome and the myotome and then the myotome splits into epimeres, which form the deep muscles of the back, and hypomeres, which form the musculature of the lateral and anterior body wall
Table 4.4
Structures developed from occipital and first two spinal sclerotomes
Origin | Process/division | Anatomical part |
---|---|---|
Neural crest cell derived | Membranous ossification | Skull vortex and calvarium |
All four occipital sclerotomes, derived from the paraxial mesoderm | Enchondrosis | Skull base |
1st and 2nd occipital sclerotomes | Clivus – basiocciput | |
3rd occipital sclerotome | Exoccipital bone | Jugular tubercles |
4th occipital sclerotome (proatlas) | Hypocentrum | Anterior tubercle of clivus |
Centrum | Dens apex | |
Apical ligament | ||
Ventral neural arch | Basion – anterior margin of foramen magnum | |
Occipital condyles | ||
Midline third occipital condyle | ||
Lateral neural arch | Cruciate ligament | |
Alar ligaments | ||
Caudal neural arch | C2 lateral mass | |
Superior portion of posterior arch of C1 | ||
Dorsal fusion of first 4 sclerotomes | Posterior margin of FM | |
Occipital bone | ||
1st spinal sclerotome | Hypocentrum | Anterior arch of C1 |
Centrum | Dens | |
Neural arch | Inferior portion of posterior arch of C1 | |
Hypocentrum | Disappears | |
2nd spinal sclerotome | Centrum | Body of axis |
Neural arch | Facets | |
Posterior arch of atlas |
The clivus and the occipital bone and, hence, the foramen magnum are derived from the four occipital somites. The first two occipital sclerotomes give rise to the basiocciput (Fig. 4.7). The tip of clivus, the anterior tubercle of the C1, the dens apex and the apical ligament are derived from the fourth occipital sclerotome, otherwise referred to as the proatlas (Menezes 1996; Gladstone and Wakeley 1925; Gasser 1976). The anterior margin of the foramen magnum, as well as the occipital condyles and the midline third occipital condyle (Fig. 4.8), arises from the ventral portion of the proatlas (Prescher et al. 1996). The cruciate ligament and the alar ligaments arise from the lateral part of proatlas. The C2 lateral mass and the superior portion of the posterior arch of the atlas develop from the caudal proatlas. The posterior rim of the foramen magnum and the occiput develop from the dorsal fusion of the first four (occipital) sclerotomes. The odontoid process and the atlas vertebra are formed from the first spinal sclerotome. The atlas shows several ossification centres in development (Keynes and Stern 1988). While the lateral masses of C2 are present at birth, complete ossification may not occur until about 3 years of age when a complete ring may then be seen. The dens is the central portion of the first sclerotome, which fuses with the axis body. The neural arch of this first spinal sclerotome proceeds to form the posterior and inferior portion of the C1 arch (Menezes 1995; Koseki et al. 1993). With further development, the hypocentrum of the second spinal sclerotome disappears, but the centrum goes on to form the body of the axis body. Division of the neural arch forms the facets and the posterior arch of the axis vertebra (Keynes and Stern 1988). In summary, most of the dens develops from the first spinal sclerotome, but the terminal portion of the odontoid process arises from the proatlas, and the most inferior portion of the axis body is formed by the second spinal sclerotome (Table 4.4).
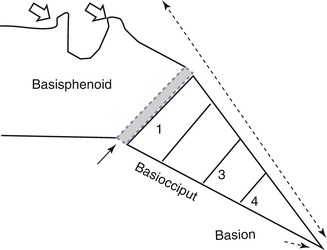
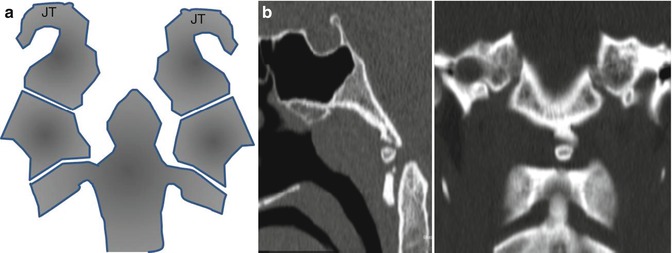
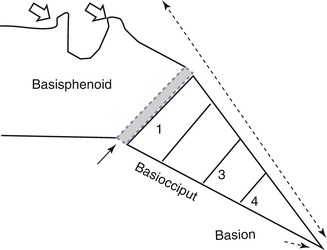
Fig. 4.7
The clivus The clivus is made up from the basisphenoid, the basiocciput and the sphenooccipital synchondrosis (closed arrow), as well as the anterior and posterior clinoids – otherwise referred to as the basi-endosphenoid (open arrows). It also includes the basion, which forms the anterior lip of foramen magnum. The basiocciput is formed by the occipital sclerotomes. The basion is formed by the 4th occipital sclerotome, or proatlas. Anomalies of the 4th occipital sclerotome are associated with Chiari malformations
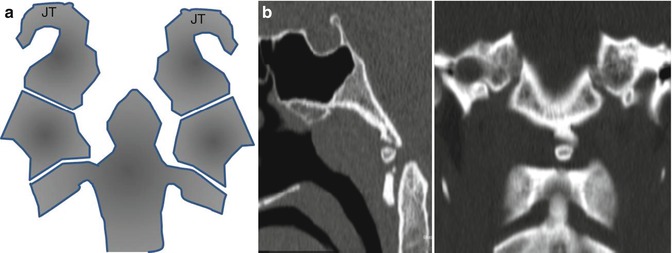
Fig. 4.8
(a) Bony structures related to the lateral boundaries of the foramen magnum. In higher vertebrates, the foramen magnum is surrounded by a ring of four bones. They arise from the third occipital sclerotome. The basioccipital bone lies in front of the opening, the two exoccipitals lie to either side, and the larger supraoccipital lies posteriorly. The jugular tubercles (JT) arise from the two exoccipital bones, which lie lateral to the foramen magnum. (b) Third occipital condyle. (a) CT sagittal. (b) Coronal multiplanar reconstruction. The third occipital condyle (condylus tertius or median occipital condyle) was first described by J.F. Meckel in 1815. It is a bony process in the anterior midline of the foramen magnum, forming a rudimentary articulation above the C1 arch. It sometimes persists into adult life
4.4.2 Development of the Skull
The skull develops by two different processes. The calvarium and facial bones develop by membranous ossification (Kessel and Gruss 1991; Christ and Wilting 1992; Dietrich and Kessel 1997) as does the occipital skull above the nuchal line, although this component is thought to arise, originally, from neural crest cells, rather than from the paraxial mesoderm. The skull base and the remainder of the occipital bone develop from a cartilaginous framework, in which deposition of bone occurs. This process is driven mainly by distorting forces generated by the developing brain. Just as remodelling of the anterior cranial fossa occurs as the prosencephalon folds down, posterior fossa expansion occurs following the growth of its neural contents. In response to the pontine and medullary enlargement, the clivus elongates at the basiocciput and lowers the front margin of the foramen magnum. Downward cerebellar displacement pushes the opisthion downward and backward. These processes result from a combination of endochondral resorption and sutural growth.
Some parts of the skull base also continue to develop later in life, in response to the growth of surrounding structures. For example, growth of the sphenooccipital and sphenopetrosal synchondrosis , along with adjacent endochondral and intramembranous ossification, results in an elongation of the clivus and the posterior skull base (Menezes 1998). This process can continue until late adolescence and will ultimately model the final shape and size of the posterior fossa.
4.4.3 Genetic Control of Mesodermal Growth
The process of segmentation at the craniocervical junction and along the spine is tightly regulated by control genes. Proteins promoted by these genes modulate the transcription of specific downstream genes, thereby controlling morphogenesis and providing specific identify for each vertebra (Lufkin et al. 1992). The main genetic groups involved in mesodermal and neuroectodermal development of the craniocervical junction are the SHH genes, for basal development, the Hox genes 7 for dorsal neural folding and tube closure and the PAX genes 8 for segmentation. Subsequent re-segmentation of the sclerotomes then occurs, to establish vertebral boundaries. This process seems to be independently controlled by two regulatory genes of the PAX family (Koseki et al. 1993).
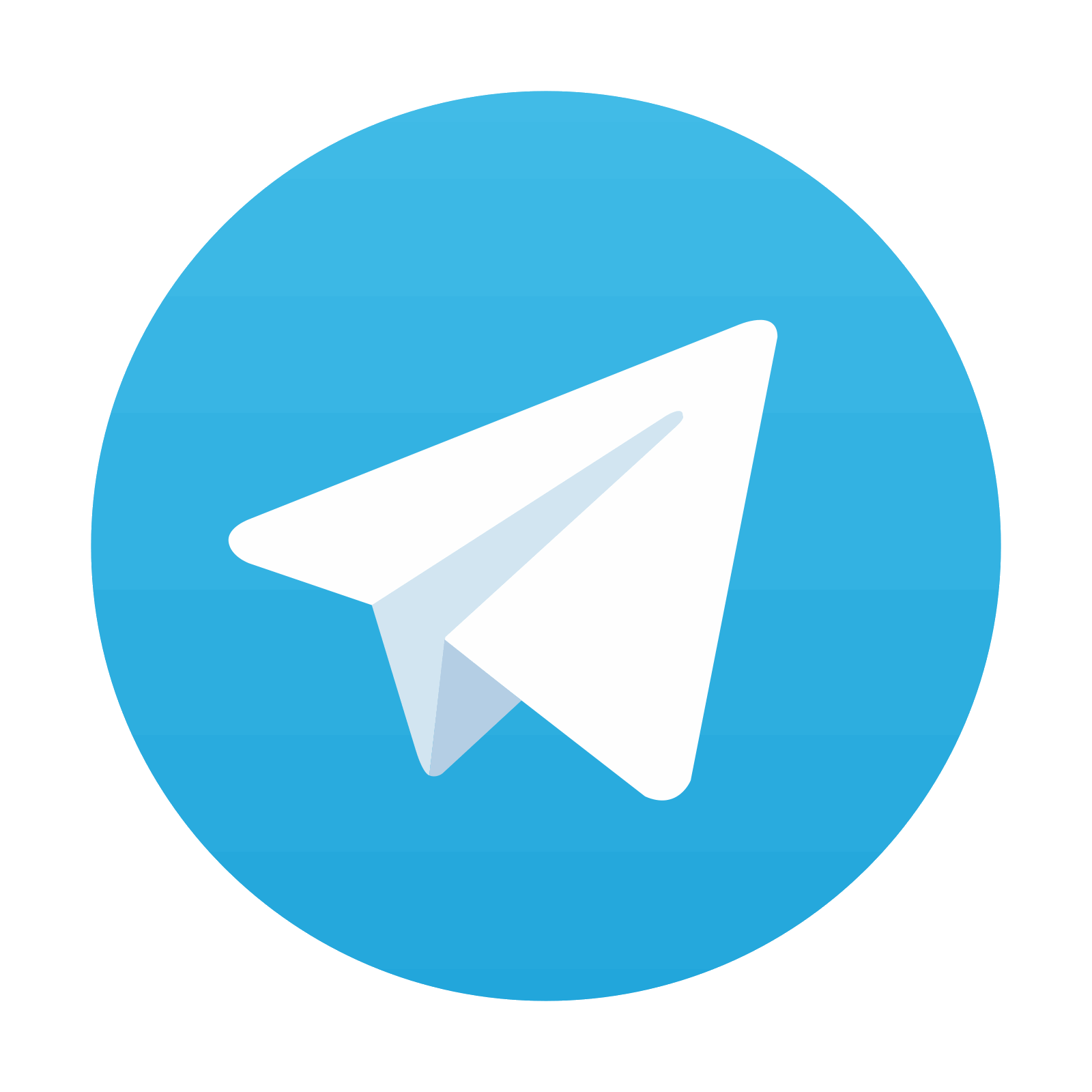
Stay updated, free articles. Join our Telegram channel
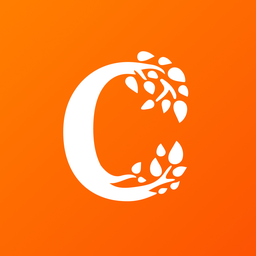
Full access? Get Clinical Tree
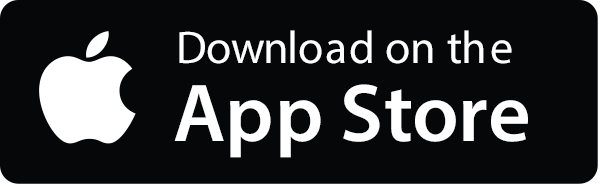
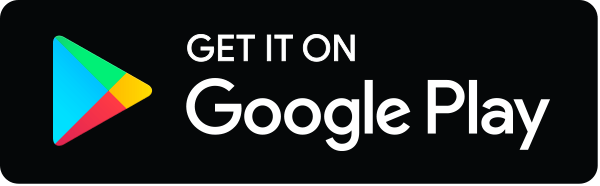