Introduction
The German psychiatrist Hans Berger made the first recording of the electric field of the human brain in 1924 in Jena. He gave this recording the name electroencephalogram (EEG). His crucial observations served as the basis for the later application of the EEG in clinical practice and promoted the fast development of clinical neurology and neurophysiology. The EEG itself represents the spontaneous electrical activity of the brain. Most brain electrical activity in a healthy individual is between 20 and 40 microvolts, although it may reach up to 100 microvolts. There are differences when measured on the scalp or directly on the brain surface. The bandwidth of the signal ranges between 0.4 and about 50 Hz and is highly variable. In contrast to spontaneous EEG background activity, evoked potentials (EPs) represent the components of the EEG that arise in response to a certain stimulus (e.g., visual, tactile, or auditory) (see Chapter 24 ). These signals are usually below the noise level and are thus not readily distinguished in the EEG background activity. Therefore one must use repetition of the stimuli and signal averaging to improve the signal-to-noise ratio to get appropriate information.
In clinical practice, the recording of EEG background activity and the application of EPs are broadly accepted. Thus EEG, including both traditional recordings and modifications such as compressed spectral analysis, is a cornerstone of neurologic diagnostics, clinical neurophysiology, and related research. Before 1996, EEGs were performed on analog machines with paper printouts. Technical advances since 2000 have led to a step-wise introduction of the EEG into neurocritical care. The era of digitization has broadened the spectrum for EEG recordings on the neurocritical care unit (NCCU) (EEG information can be recorded continuously and stored digitally). EEG and EPs have become common and valuable tools in the NCCU. In particular, different EEG-derived parameters can help predict individual clinical prognosis and outcome. Continuous EEG (cEEG) allows real-time monitoring of the underlying cerebral physiology noninvasively and cost effectively in critically ill patients, and it can be used to gauge the efficacy of certain medications or therapeutic maneuvers. For example, cEEG can be used for seizure diagnosis in comatose patients after traumatic brain injury (TBI), detection of delayed cerebral ischemia (DCI), after subarachnoid hemorrhage (SAH), and titration of therapies for status epilepticus or barbiturate-induced coma among others. However, the integration of EEG recording into the environment of neurocritical care requires a refined technical setup and highly trained technical and medical staff. These necessities require a mutidisciplinary team. This chapter focuses on the application of intermittent (iEEG) or cEEG monitoring in the NCCU.
Physiologic Electroencephalogram Background Activity
In a healthy individual, typical EEG background activity consists of a well-organized and predictable spectrum of waveforms. However, numerous factors may influence the frequency and organization of these waveforms. There exist ultra-slow and ultra-fast frequencies that in general do not play a significant role in clinical practice in the intensive care unit (ICU). However, ultra-fast frequencies can be clinically useful in predicting outcomes from epilepsy surgery. Very low-frequency changes usually are filtered out and although they may be observed in patients in coma or preterminal conditions, they usually do not provide clinically useful information. In clinical practice the frequency range of the EEG is broken down as follows:
Delta rhythm: 0.1 to 3.5 Hz
Theta rhythm: 4 to 7.5 Hz
Alpha rhythm: 8 to 13 Hz
Beta rhythm: 14 to 30 Hz
Gamma rhythm: >30 Hz (often filtered out on scalp EEG)
Several aspects are essential for EEG interpretation: (1) frequency, (2) amplitude, (3) rhythmicity, (4) morphology, and (5) spatial distribution. These aspects are highly variable under normal conditions and show a strong dependence on numerous factors such as age, level of consciousness (alertness), and physical or mental activity. In addition, many external or intrinsic stimuli can influence EEG background activity.
Pathologic Electroencephalogram Activity
EEG background activity is very sensitive to changes in brain homeostasis and physiology. EEG may show manifestations of numerous conditions, including metabolic encephalopathy, systemic hypoxia, brain trauma, ischemic and hemorrhagic stroke, brain tumor, and infectious disease to name a few.
Any change in electrical brain activity, either focal or generalized, that leads to a failure of local compensation may cause epileptic discharges. The extent and duration of EEG alterations are highly variable. An electrographic seizure is defined as a pattern lasting more than 10 seconds with (1) epileptiform waveforms at 3 Hz or greater, (2) epileptiform waveforms at less than 3 Hz with clear evidence of evolution in time or space and (3) evidence of clinical or electrographic improvement following administration of an antiepileptic drug (AED. Fig. 25.1 ). Furthermore, a seizure is likely to be present if the pathologic EEG is characterized by an evolution in frequency, morphology, and location.

Types of Seizures, Related Phenomena, and Differential Diagnosis
In the clinical setting many seizures are easily recognized and therefore amenable to direct intervention and treatment. Convulsive seizures (either primary or secondarily generalized) are usually clinically obvious and do not require an EEG for diagnosis. However, many focal seizures are associated with few or no outward clinical manifestations. These seizures, which are referred to as nonconvulsive seizures or subclinical seizures require an EEG for their diagnosis, including among patients with impaired consciousness in whom clinical diagnosis of a seizure may be difficult. In this group of patients EEG also can help assess status epilepticus (SE), nonconvulsive status epilepticus (NCSE), and seizure-like activity such as periodic epileptiform discharges (PEDs) or generalized periodic epileptiform discharges (G-PEDs).
SE is a neurologic emergency with high mortality and morbidity. There are several types of SE, and consequently it has several definitions. The International League Against Epilepsy defines SE as “a seizure that persists for a sufficient length of time or repeated frequently enough that recovery between attacks does not occur.” SE can be subdivided based on clinical and EEG criteria into generalized convulsive status (tonic-clonic, tonic, clonic, and myoclonic), generalized nonconvulsive (absence) status, elementary partial status (with several subtypes), and complex partial status. Official definitions have not included the exact duration of time, but based on animal studies that demonstrate significant brain damage after 30 minutes, convulsive status epilepticus (CSE) traditionally is considered as one continuous unremitting seizure that lasts greater than 30 minutes. CSE may be observed in between 1% and 30% of ICU patients. However, it is impractical and unsafe to wait 30 minutes before initiating treatment and because it is rare for generalized convulsive seizures to spontaneously cease after 5 minutes, some suggest that a duration of 5 or 10 minutes may be a reasonable operational definition. NCSE is defined as an impairment of consciousness or behavior from baseline state for at least 30 minutes without convulsive movements, and the presence of one or more of the following: (1) repetitive focal or generalized epileptiform activity (spikes, sharp waves, spike-and-wave, sharp-and-slow wave complexes) or rhythmic theta or delta activity at more than two per second; (2) the EEG patterns described in 1 at less than one per second, but with improvement after intravenous (IV) AED injection (e.g., a benzodiazepine); or (3) a temporal evolution of epileptiform or rhythmic activity greater than 1 Hz with change in location or frequency over time and normalization (either clinically or electrographically) in response to AED administration. If a patient experiences frequent recurrent seizures without a clear return to baseline mental status between seizures, then he or she is in NCSE by definition. Many of the patients with NCSE have subtle motor manifestations; these manifestations may be focal or generalized. NCSE may be classified according to patient age or subdivided into: (1) complex partial SE (CPSE) or (2) generalized nonconvulsive SE.
Nonconvulsive seizures (NCS) are common in critically ill patients, and many studies suggest that the majority of seizures in these patients have limited clinical manifestations and can only be recognized with EEG monitoring. After primary brain injury, NCS occur in 11% to 55% of patients. The variable prevalence of NCS reported in the studies may reflect different injury types and different levels of sedation present during the EEG recordings. The criteria for diagnosis are listed in Table 25.1 . The clinical spectrum of NCS is broad, and without prolonged monitoring the majority of NCS will not be identified. However, it is unclear what constitutes an adequate duration of monitoring. Several studies suggest that the continuous monitoring of EEG background activity is necessary for at least 48 hours to detect 85% of the CSE and NCSE in comatose ICU patients. Thus the latency for the detection of NCSE or CSE might be as long as 48 to 72 hours, or even up to 120 hours.
Any pattern lasting at least 10 seconds satisfying any one of the following three primary criteria:
|
Up to 50% of individuals with a history of generalized tonic-clonic seizures who fail to return to baseline despite adequate treatment have evidence of NCSE on EEG. There is evidence that NCSE may exacerbate neuronal injury. For example, NCSE is known to lead to significant hippocampal atrophy after TBI indicative for severe neuronal injury. It can also exacerbate midline shift in intracerebral hemorrhage (ICH) patients. In addition, nonconvulsive electrographic post-traumatic seizures have been observed to result in increases in ICP and microdialysis markers of cellular distress (e.g., lactate pyruvate ratio or glutamate).
A variety of other periodic EEG patterns can be observed in critically ill patients such as triphasic waves, periodic lateralized epileptiform discharges (PLEDs), or burst suppression. PLEDs are considered traditionally an interictal epileptiform disturbance ( Fig. 25.2 ). They are observed most frequently with acute focal destructive lesions (e.g., infarct, infection, or tumor). The presence of PLEDs may predict a delayed time to first seizure among ICU patients who undergo cEEG monitoring; that is, if PLEDs are present a longer duration of cEEG may be indicated. A spontaneous burst-suppression pattern indicates severe brain dysfunction particularly in comatose patients. This condition is associated with bursts of cortical activity that alternate with near complete suppression of background activity. The most common context in which burst suppression occurs is in pharmacologically induced comas for the control of elevated ICP (see following text) or refractory status epilepticus. However, a nonpharmacologically induced burst-suppression pattern may be seen in patients with severe diffuse brain injury, particularly in severe diffuse anoxic brain injury. In addition, focal or generalized nonseizure activity, like the aforementioned rhythmic or periodic sharp transients, is frequently observed in critically ill patients. The incidence of G-PED or PLED ranges between 50% and 58%. Patients with PEDs are at high risk of seizures. G-PEDS represent a rare phenomenon that was observed in 37 of 3000 EEG recordings only in one center. In this series, 89% of 37 patients had either a myoclonic seizure (35.2%), a generalized convulsive seizure (GCS) (21.6%), or generalized status epilepticus (GSE) (32.4%) within 48 hours of the detection of the G-PEDs. The occurrence of G-PEDS and its variants is associated with subacute sclerosing panencephalitis (SSPE) and sporadic Creutzfeldt-Jakob disease but most frequently observed acutely in patients with diffuse anoxic brain injury.

The application of high-frequency recording by means of subdural grid electrodes allows the detection of self-propagating waves of neural and glial depolarization. This slow progressing wave that spreads at 2 to 5 mm/minute over the cortex is known as cortical spreading depression (CSD). In the normal brain the EEG shows a period of transient depression of cortical electrical activity that is associated with an increase in regional CBF and oxygen delivery (intact neurovascular coupling). This phenomenon has a multifactorial etiology and is known to occur in migraine. Under pathologic conditions, however, neurovascular coupling is impaired and the depolarization wave causes a reduction in rCBF (spreading ischemia, neurovascular uncoupling). The increased energy demands imposed by CSD may worsen neuronal injury under these conditions. Thus after aneurysmal SAH the number and duration of clusters of CSD are temporally linked to the development of delayed infarct. The number of clusters is highly variable and may last between 3 and 60 hours.
Electroencephalogram Methodology
Duration of Electroencephalogram Monitoring
The occurrence and time course of seizures, NCS, NCSE, and PLEDs among others is highly variable and disease or pathology dependent. Routine iEEG recordings, which last less than 30 minutes, fail to capture a seizure in more than half of all seizing patients. However, up to 50% of the seizures occur within the first 60 minutes of EEG recording and 80% of comatose patients have seizures within 24 hours of monitoring. Thus in comatose patients, exclusion of NCS or NCSE requires at least 24 to 48 hours of recording time, whereas the duration of routine iEEG should be at least 30 to 60 minutes long for seizure detection. When EEG is used to detect ischemia and in particular DCI after aneurysmal SAH, monitoring should continue for the whole period of risk, up to 14 days after insult.
Electrode Placement and Montage
Scalp pathology or other cranial injuries can limit electrode placement and data quality. For monitoring of generalized activity the standard 21 electrodes according to the 10-20 system may be adequate. A few studies suggest 10 electrode positions and a structured 14-channel montage with referential and bipolar derivations for monitoring in patients with TBI. For cEEG monitoring Labar et al. successfully used 5 electrodes and 2 channels to analyze trends in compressed spectral arrays in SAH patients. However, it is generally accepted that reducing the number of electrodes limits resolution of waveform morphology and distribution. Therefore focal abnormalities may be more difficult to recognize. Furthermore, full electrode configuration improves the ability to distinguish brain signals from artifacts, aids in spatial localization of pathologic activity, and provides redundancy.
Alternative methods are amplitude-integrated EEG (aEEG) monitoring using two electrodes for seizure detection, a technique used in purpose-built devices that are common in neonatal ICUs. Most studies suggest a sensitivity of about 60%, but in some studies using this technique a third or fewer of seizures identified using conventional electrode arrangements are detected with aEEG. Similarly, a six-electrode configuration placed at the hairline and compared to a full electrode montage may miss a third of seizures and up to one half of PLEDs. Because ictal arrhythmias, including ictal asystole and ictal bradycardia, are relatively common phenomena, a channel dedicated to electrocardiographic monitoring, preferably using two noncephalic electrodes, is nearly always needed and may reveal important changes in the cardiac rhythm that may be seen in association with some seizures. A noncephalic electrocardiographic channel can help distinguish between certain artifacts and rhythmic EEG discharges. In daily practice, skin breakdown can be a problem, particularly when cup electrodes are used for prolonged periods. Alternatively needle electrodes can be used, but there are risks associated with this such as needlesticks or broken subdermal wires. Recently, intracortical electrodes that are placed stereotactically into deep brain regions have been used in clinical research. Using this approach, Stuart et al., were able to demonstrate the potential value of depth electrodes to detect DCI associated with vasospasm in poor-grade SAH patients.
Data Analysis
Recording of EEG background activity requires the inspection of the raw EEG. The visual analysis remains the standard method of EEG assessment. It is the best method to recognize seizures and to detect reasonably rapid (seconds to minutes) changes in EEG content on an expert level. The obvious disadvantages are the time efforts needed for data review and the low sensitivity to gradual trends in the EEG (loss of faster frequencies or changes in variability of frequency content). In addition, this method is highly subjective, and interpretation of EEG patterns can vary between different electroencephalographers. Therefore visual analysis of the raw EEG signal alone limits the application and beneficial impact of cEEG monitoring technology in the ICU. Various approaches to process the raw EEG improve the diagnostic accuracy and cut down the time needed for data interpretation. The aEEG method depicts time-compressed and rectified EEG amplitude derived from raw EEG on a semi-logarithmic scale, whereas frequency domain methods of EEG present an alternative approach of signal simplification. The aEEG provides an accurate measure of EEG background activity that can be useful for clinical application. Color density spectral array (CDSA) applies fast-Fourier transformation (FFT) to convert raw EEG into a time-compressed and color-coded display ( Fig. 25.3 ). Clinical applications of quantitative EEG such as CDSA and related techniques include monitoring the depth of sedation, detection of cerebral ischemia, and identification of seizures in adults, children, and neonates. The use of cEEG may be most useful in patients that require long-term monitoring, for example, after poor-grade SAH when patients are at risk for both seizures and ischemia. Claassen et al. reported that 19% of patients who had cEEG after poor-grade SAH (n = 108) had seizures, and 95% of these seizures were NCS without any detectable clinical correlate. The application of quantitative analysis to the cEEG (relative alpha variability, post-stimulation alpha-to-delta ratio [ADR]) enhances its use in detection of DCI and increases its sensitivity for ischemia detection. However, this does not improve the sensitivity for seizure detection, but its use can accelerate the process of screening the EEG for seizures. When using quantitative cEEG, raw EEG data should be available for individual review. The application of video EEG recordings (vEEG) can help increase the likelihood for seizure detection on the ICU, particularly in patients with suspected NCSE.

Electroencephalogram Artifacts and Artifact Detection
Typical artifacts of the EEG are either non-neural physiologic activities of the subject or caused by external technical problems. Physiologic artifact sources are eye blinks, eye movements, muscle activity in the vicinity of the head (e.g., face muscles, jaws, tongue, neck), heartbeat, pulse, and Mayer waves. Most problematic in clinical practice are ocular (electro-oculogram [EOG]) and muscle (electromyogram [EMG]) artifacts. EOG activity is caused by lateral eye movements or by eye blinks (f = 20 times/min). This results in low-frequency activity most prominent over the anterior head regions (f max = 4 Hz), whereas EMG activity usually shows as high-frequency activity (>20 Hz), with a wide range of amplitudes. A wide variety of technical artifacts can be seen, including induction artifact, electrostatic artifact, capacitative artifact, and 60 Hz artifact. In particular, the presence of an electrical appliance running on an AC circuit can create a 60-Hz artifact. Artifact rejection remains challenging in practice where hand-optimized, semiautomatic, and fully automatic approaches exist. Semiautomatic approaches, although less time consuming, require user interaction for ambiguous or outlier components. Fully automated methods are proposed for the classification of eye artifacts but are used only in some nonstandardized research protocols. These methods, although robust for the detection of eye artifacts, are not easily usable for non–eye artifacts and may require the additional recording of the EOG. Future developments include EEGLAB plug-ins and support vector machine (SVM)–based systems that in a fully automatic mode recognize and reject major artifacts like eye blinks, eye movements, and heartbeats; the detection of muscular or more subtle artifacts has not been reported so far.
Indications for Electroencephalogram Monitoring
General Considerations
EEG is increasingly recognized as a useful tool to detect seizures and seizure-like activity in different pathologies involving the central nervous system (CNS) and to serve as a diagnostic tool for coma of unknown etiology. In addition, EEG recordings help monitor and direct therapeutic maneuvers in the NCCU. This includes the monitoring of the burst-suppression pattern during treatment of refractory intracranial hypertension, the monitoring of treatment efficacy for NCSE and CSE, and the detection of DCI.
Traumatic Brain Injury
In TBI, seizures are identified more frequently when EEG is performed than through clinical observation. However, the exact incidence varies according to the methodology used—AEDs, sedatives, and duration of monitoring. For example, in a prospective study of 94 severe TBI patients who underwent cEEG (on average for 7 days), Vespa et al. observed seizures in 22% of the subjects, half of which were NCS: 50% of seizures occurred within day 1, despite phenytoin prophylaxis. Brain contusions and skull fractures were not associated with increased seizures. When no antiepileptic prophylaxis was given, Ronne-Engstrom and Winkler observed NCS in 28% and NCSE in 11% of TBI patients (n = 70) who had cEEG monitoring more than 24 hours. NCS were associated with extracerebral or intracerebral hematomas and older age (>60 years). The majority of these events lasted less than 30 minutes and all responded to benzodiazepines and phenytoin. On the other hand, Amantini et al. observed far fewer seizures in TBI patients (3%). These differences may be associated with use of a much greater dose of medications such as midazolam, propofol, thiopental, or a combination, that can have antiseizure activity.
EEG recordings have been used to predict outcome in TBI patients. Several factors are associated with outcome, including (1) an increase or decrease in the absolute and relative amplitudes in the alpha and theta bands in the EEG can help differentiate survivors from nonsurvivors ; (2) a delta EEG pattern is associated with death and poor functional outcome ; (3) a reduced percentage of alpha variability is associated with poor prognosis ; and (4) the presence of EEG reactivity to painful stimuli is associated with a good outcome. However, prognostic errors based on EEG may be observed in 20% of patients.
Aneurysmal Subarachnoid Hemorrhage
The incidence of seizures after aneurysmal SAH (aSAH) varies from 4% to 24%. Most cases occur at the time of aneurysm rupture or shortly after the initial hemorrhage. Risk factors for seizures include a history of epilepsy, middle cerebral artery aneurysms, a cerebral infarct, and an intracerebral hematoma. NCS also are identified after SAH. The incidence varies with the method of detection. For example, the incidence of NCS is 3%, when using repeated standard (i.e., iEEG). When using cEEG, and in particular for more than 24 hours, seizures are detected in 19% of patients, and the majority (95%) of them are NCS. Up to 8% of patients have NCSE, despite antiepileptic prophylaxis (fosphenytoin). Risk factors for NCSE include (1) older age, (2) female sex, (3) poor-grade SAH, (4) the amount of cisternal blood, (5) hydrocephalus, and (6) the presence of structural brain lesions including focal or global cerebral edema. NCSE can be refractory to therapy, and many of these patients have a poor outcome. In many of these series cEEG was used in patients with convulsive seizure activity, unexplained neurologic deterioration, or persistent and unexplained coma, but NCS are still common even without any detectable clinical correlate.
EEG including iEEG, aEEG, and cEEG recordings can help detect DCI. For example, Rivierez et al. studied 151 SAH patients on day 1 and day 5 after SAH with standard EEG, and found that patients (n = 78) with bilateral bursts of slow waves were more likely to have DCI. Artifacts and the need for manual postprocessing of the raw data can limit the use of EEG to detect DCI. However, quantitative EEG analysis (qEEG) holds promise as a method to detect DCI ( Fig. 25.4 ). A decrease in the ADR extracted from the cEEG shows a strong association with DCI in poor-grade SAH patients. The median decrease of ADR for patients with DCI was 24%, whereas an increase of 3% was observed in patients without DCI associated with vasospasm. In a cohort of 32 mainly good-grade patients monitored with cEEG and trending of a quantitative measure, the relative alpha variability, Vespa et al. found that in all 19 patients with angiographically proven vasospasm, relative alpha variability was significantly decreased and preceded the diagnosis of vasospasm by 2 to 3 days. Table 25.2 lists the relation between EEG changes and the corresponding changes in regional cerebral blood flow (rCBF); these changes can be used to help differentiate reversible from irreversible ischemia. When the EEG waveform pattern is suppressed, rCBF is less than 8 to 10 mL/100 g/minute and indicates irreversible tissue damage.

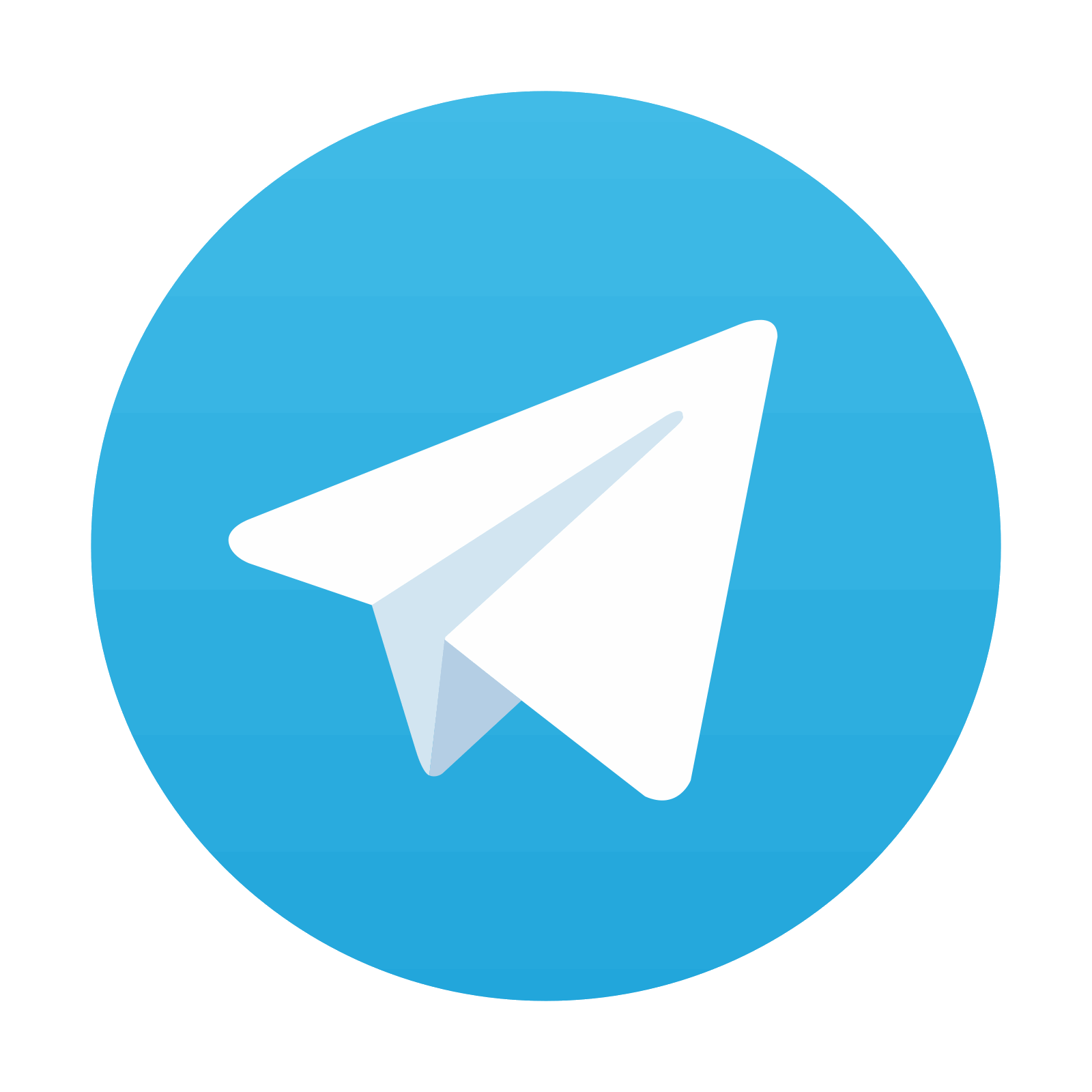
Stay updated, free articles. Join our Telegram channel
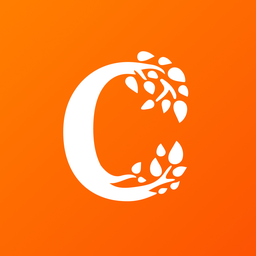
Full access? Get Clinical Tree
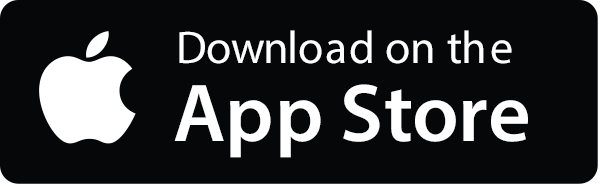
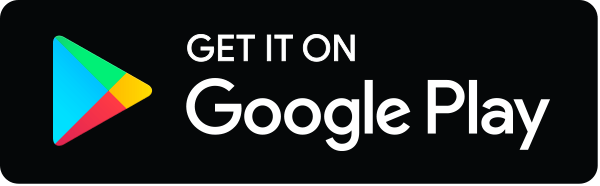
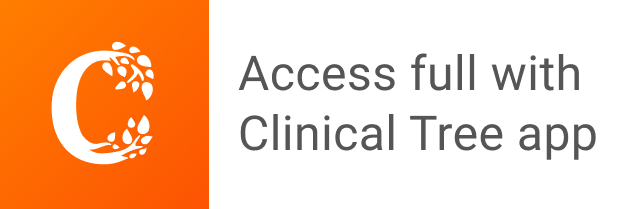