Tests of neuromuscular transmission are used most often to detect the abnormalities characteristic of myasthenia gravis. Although myasthenia is not a common disease, it is often included in the differential diagnosis of patients with symptoms of weakness and fatigue who are referred for electrodiagnostic studies. Much less common diseases with abnormal neuromuscular transmission are Lambert–Eaton myasthenic syndrome (LEMS) and botulism. Neuromuscular transmission may also be abnormal in primary nerve or muscle disease and may be demonstrated by sensitive electrodiagnostic tests, such as single-fiber electromyography (EMG). Electrophysiologic tests of neuromuscular transmission require experience and careful attention to detail. Information from these tests complements that from tests of nerve conduction and needle electromyography.
Normal neuromuscular transmission
The neuromuscular junction consists of the motor nerve terminal, a bulbous structure at the end of the motor nerve; the muscle endplate, with its folded postsynaptic membrane; and the synaptic cleft, which separates these two structures. In most human muscles, there is one endplate on each muscle fiber, located approximately halfway along the length of the fiber. Synaptic transmission from nerve to muscle involves a complex series of electrical and chemical reactions ( Fig. 17-1 ).

The neuromuscular transmitter acetylcholine (ACh) is synthesized in the motor nerve and stored within vesicles in the nerve terminal. The amount of ACh in a vesicle constitutes a basic unit or “quantum” of transmitter. When the motor nerve is activated voluntarily or by electrical stimulation, a wave of depolarization travels distally to the nerve terminal from which, via a complex mechanism that requires the presence of calcium in the extracellular fluid, there is near-simultaneous release of many ACh vesicles into the synaptic cleft. The neurotransmitter crosses the synaptic cleft to interact with specific receptors on the tips of the folded postsynaptic muscle membrane. Ion channels in the endplate membrane are opened by the interaction of ACh and receptor, allowing Na + to move into the muscle. This produces a local depolarization at the endplate, the endplate potential (EPP). If the EPP is sufficiently large, depolarization spreads to the periendplate region and then radially to both ends of the muscle fiber, with subsequent excitation-contraction coupling and muscle contraction. After interaction with the receptor, the ACh is broken down to choline and acetate by acetylcholinesterase (AChE) on the endplate membrane. The choline is taken up by the nerve and incorporated into newly synthesized ACh.
During stimulation of the motor nerve, the number of ACh quanta released from the nerve terminal varies. At stimulation rates greater than 0.1 Hz, progressively fewer quanta are released by the first four or five nerve discharges of a train (“depression”), after which the mean amount of ACh released per impulse becomes relatively constant, with some variation around the mean value ( Figs. 17-2 and 17-3 ).


Following muscle activation, there is a period of “facilitation,” during which each nerve impulse releases more ACh than before activation. Facilitation lasts for 30 to 60 seconds and is followed by a period of “postactivation exhaustion,” during which less ACh is released by each nerve impulse. Exhaustion is maximal 2 to 5 minutes after the end of activation.
The ratio of the EPP amplitude to the threshold for action potential initiation is called the “safety factor” of neuromuscular transmission. In normal muscle, the safety factor is great enough to ensure that each nerve impulse is followed by muscle contraction (see Fig. 17-3 ).
The amount of ACh released by a nerve impulse depends on the interplay among these different processes, which have no clinical or electromyographic manifestations unless the safety factor is reduced. When neuromuscular transmission is impaired, however, the action potential threshold in some muscle fibers may be exceeded only by the first few impulses of a train of stimuli, and transmission of the later impulses fails (see Fig. 17-3 ). This manifests electromyographically as a decrementing muscle response to repetitive nerve stimulation ( Fig. 17-4 ). Postactivation exhaustion also may be seen when neuromuscular transmission is abnormal ( Fig. 17-5 ). Postactivation facilitation is seen most dramatically when there is a presynaptic block, as in LEMS ( Fig. 17-6 ).



Neuromuscular transmission may be blocked at many sites (see Fig. 17-1 ). For example, hemicholinium blocks the uptake of choline necessary for the synthesis of ACh and leads to depletion of ACh stores in the nerve terminal; botulinum toxin blocks the release of ACh from the nerve terminal; curare interferes with the interaction of ACh and receptor; and cholinesterase inhibitors block the action of acetylcholinesterase, causing ACh to accumulate at the endplate, producing depolarization block.
Variation in the amplitude of endplate depolarizations causes the EPPs to reach threshold at slightly different times ( Fig. 17-7 ). This variation in time between nerve impulse and generation of the muscle action potential is called the neuromuscular jitter. When the safety factor of neuromuscular transmission is reduced for any reason, be it a presynaptic abnormality of quantal release or postsynaptic block of the receptors, the neuromuscular jitter increases; thus, jitter is a reflection of the safety factor of neuromuscular transmission.

Release of ACh from the motor nerve and the response of the muscle to known amounts of this transmitter have been measured in biopsied muscle to define the pathophysiology of diseases in which neuromuscular transmission is abnormal (e.g., myasthenia gravis and LEMS). Microelectrodes are inserted near the endplate to measure intracellular potentials. Single quanta of ACh constantly are being released spontaneously from nerve terminals, and these produce transient depolarizations of the muscle endplate called miniature endplate potentials (MEPPs). The MEPP amplitude is a measure of the amount of ACh in each quantum. Intracellular recordings in muscle biopsies have shown that MEPP amplitude is reduced in myasthenia gravis because of decreased sensitivity of the postjunctional muscle membrane to applied ACh. In LEMS, such studies have demonstrated that the endplate responses to ACh are normal, but fewer quanta are released from the nerve.
In the developing neuromuscular junction, clustering of ACh receptors (AChRs) is dependent upon muscle-specific tyrosine kinase (MuSK) and rapsyn. In response to agrin, a glycoprotein secreted from motor nerve terminals, MuSK induces clustering of AChR on the postjunctional membrane. In mature, innervated muscle MuSK is essential in maintaining normal AChR clustering. Circulating antibodies to MuSK have been found in up to 50 percent of patients with generalized myasthenia gravis who do not have antibodies to the AChR. (See the section on myasthenia gravis later in the chapter.)
Repetitive nerve stimulation
The most commonly used electrodiagnostic test of neuromuscular transmission is repetitive nerve stimulation. Progressive reduction or disappearance of visible muscle contraction during faradic stimulation in myasthenia gravis was described by Jolly in 1895. He called this a “myasthenic reaction.” Harvey and Masland described the decrementing muscle electrical response to repetitive nerve stimulation that is the basis of the test in current use.
In this test, compound muscle action potentials (CMAPs), which reflect the number of activated muscle fibers, are recorded during repetitive stimulation of the motor nerve. Repetitive nerve stimulation produces a decrementing pattern in a train of CMAPs if progressively fewer muscle fibers respond to nerve stimulation (see Fig. 17-3 ). This is the typical finding when the number of blocked endplates increases during repetitive nerve stimulation as a result of depletion of the readily releasable transmitter stores. However, a decrement may not be seen if many endplates are blocked at rest or if the total number of blocked endplates does not change during repetitive nerve stimulation.
Recording Technique
Technically excellent recordings are necessary for minimizing artifacts. The recording electrodes should be placed so that there is an initially sharp negative (upward-going) deflection of the CMAP, indicating that the active (G1) electrode is over the motor point of the muscle. The reference (G2) electrode should be over a distal point where minimal activity is recorded. It may be necessary to immobilize the appropriate joints if movement artifact interferes with measurements of the CMAP. The stimulating and ground electrodes should be positioned to minimize stimulus artifact. The low-frequency filter should be 10 Hz or lower for most muscles, but testing of proximal muscles (e.g., biceps or deltoid) requires settings as low as 2 Hz. The high-frequency filter should be 5 kHz or higher. The stimulus duration should be no longer than is necessary for ensuring maximal stimulation during the entire testing session. A setting of 0.1 msec is usually adequate when surface electrodes are used for stimulation, but this is determined empirically for each stimulation site. If stimulation is performed with needle electrodes inserted near the nerve, shorter-duration, lower-intensity stimulation pulses are adequate and are less painful. With either type of stimulating electrode, the stimulus intensity should be 10 to 25 percent greater than that necessary for activating all muscle fibers. This helps to ensure that all responses are maximal during trains of stimuli.
Muscle Temperature
The decremental response in myasthenia gravis and LEMS is less when the muscle is cool ( Fig. 17-8 ). In myasthenia gravis, it is common to see a normal response to repetitive nerve stimulation when the surface temperature over the muscle is below 30°C (which is common in unwarmed hand muscles) and then to find a significant decrement after the muscle has been warmed. Hand or foot muscles should be warmed to a surface temperature of at least 34°C. Cooling is not a factor in proximal or facial muscles, which need not be warmed. In myasthenia gravis, the decrement is greater at a surface temperature of 44°C than at 32°C, but it is not clear that heating above physiologic temperatures increases the sensitivity of repetitive nerve stimulation testing.

Stimulation Technique
A decremental response in myasthenia gravis is demonstrated best at stimulation rates of 3 to 5 Hz, and the decrement increases with the stimulation rate, up to 10 Hz ( Fig. 17-9 ). At higher stimulation rates, artifacts caused by movement are common and decrements up to 50 percent have been reported in normal muscle at rates greater than 10 Hz.

The CMAP size may increase during high-frequency nerve stimulation or after voluntary activation. This is called potentiation . Potentiation may result from an increase in the number of activated muscle fibers (facilitation) or in the amplitude or summation of action potentials of individual muscle fibers (pseudofacilitation) (see Figs. 17-4 and 17-10 ). Each nerve depolarization releases calcium into the periterminal space, which increases the local concentration of calcium for 100 to 200 msec. If another depolarization occurs during this time, the higher calcium concentration increases the number of ACh quanta released. When neuromuscular transmission is impaired, this greater ACh release may improve synaptic transmission briefly, producing facilitation. Marked facilitation is characteristic of presynaptic blockade, but is also seen occasionally in myasthenia and during poisoning with curare, which has a predominantly postsynaptic action. Thus, facilitation in itself does not always indicate a presynaptic abnormality.

Pseudofacilitation has been attributed to increased synchronization of propagation velocities of muscle fiber action potentials or to shortening of muscle fiber length, particularly when the muscle is tested without fixation. In normal muscle, pseudofacilitation may increase the CMAP amplitude by as much as 50 percent during stimulation at rates up to 50 Hz. This can mask a decrementing response or may be mistaken for true facilitation. The duration of the CMAP decreases during pseudofacilitation, and the area of the waveform therefore remains relatively constant (see Fig. 17-10 ). Measuring the duration of the CMAP will determine how much the waveform has shortened during activation, and will indicate whether pseudofacilitation has contributed to at least some of any potentiation.
Measurement Technique
The size of the CMAP may be assessed by measuring the peak-to-peak or negative-peak amplitude. Many technical problems that arise during repetitive nerve stimulation can be detected if the CMAP waveforms are observed at a display setting of 50 to 100 msec per screen during stimulation. Movement of the stimulating electrode or the joint may produce changes in CMAP size that do not correspond to patterns expected in disease (see Fig. 17-4 ). In myasthenia gravis, the typical pattern is a progressive decrement from the second through the fourth or fifth response, with some return toward the initial CMAP size during the subsequent responses (see Figs. 17-4 and 17-5 ).
Changes in CMAP size are quantified by calculating the percentage change in amplitude between the first and the fourth (or fifth) responses of a train of stimuli, using the following formula:
D 4 ( or 5 ) = V 4 ( or 5 ) − V 1 V 1 × 100
- 1.
Reproducibility . The same decrement should be seen when the stimulation is repeated after a period of rest.
- 2.
Envelope shape . Changes in CMAP amplitude during a train of stimuli should conform to a pattern seen in disease, without sudden or irregular variations between consecutive responses (see Fig. 17-4 ).
- 3.
Activation cycle . The changes induced by activation (discussed in the following section) should conform to an acceptable pattern (see Fig. 17-5 ).
- 4.
Response to edrophonium (Tensilon) . Administration of intravenous edrophonium usually reduces or obliterates the decrement in myasthenia gravis.
Measurements of the CMAP amplitude during high-frequency stimulation may give variable and inconsistent results, and it has been recommended by some that the CMAP area, rather than amplitude, be measured to assess facilitation. However, area measurements are affected by the position of the reference electrode, repolarization, and muscle fiber fatigue, and are markedly dependent on filter settings. In most cases, the results from area and amplitude measurements are concordant; any discrepancy should lead to a search for technical problems.
Activation
Facilitation may be induced either by high-frequency nerve stimulation (20 to 50 Hz) for 10 seconds or by a brief maximal voluntary contraction of the tested muscle. The latter is much less painful. Trains of low-frequency stimuli are given at the end of activation to demonstrate facilitation and then every minute or, later, every other minute for up to 10 minutes to demonstrate postactivation exhaustion. Voluntary muscle contraction is maintained for 10 seconds to demonstrate facilitation, as when testing for LEMS, or for 60 to 90 seconds to demonstrate exhaustion. In LEMS, the postactivation stimulus must be given as soon as possible after the end of activation because facilitation persists for only a short time.
Muscle Selection
In myasthenia gravis, proximal muscles usually are affected earlier and more severely than are distal muscles, and there is usually a higher diagnostic yield with repetitive nerve stimulation studies of proximal or facial muscles. To obtain the maximal diagnostic yield, it is necessary to test several muscles, including those most likely to be involved in the individual patient.
Hand Muscles
Hand muscles are the most convenient to test. Recordings usually are made from the hypothenar muscles while the ulnar nerve is stimulated or from the thenar muscles while the median nerve is stimulated. The hand can be immobilized by specially designed clamps or straps, but it is usually adequate for the examiner to hold the patient’s hand firmly on the examining table. Activation is produced by having the patient contract the tested muscle against resistance. The temperature should always be monitored in these muscles.
Biceps
The biceps is tested by stimulating the musculocutaneous nerve in the axilla with the patient supine and the arm supinated on the examining table or armrest. Recording electrodes are placed on the belly and tendon of the muscle in a position that gives a monophasic CMAP. Stimulation can be performed with surface electrodes pressed firmly against the posterior border of the short head of the biceps within 1 inch of the inferior edge of the axillary fold. Care should be taken to avoid stimulating other nerves in the axilla. The position of the stimulating electrode must be kept constant while trains of stimuli are applied. The arm must be restrained to prevent movement, which can produce artifacts in the recordings and can dislodge the stimulating electrode. Immobilization can be achieved by the examiner holding the forearm firmly against the supporting surface. Activation is produced by having the patient flex the elbow against resistance. This test is uncomfortable, especially if prolonged or high-frequency stimulation is used. Stimulation with a needle electrode inserted near the nerve reduces discomfort, and the needle electrode is less likely than a surface electrode to be dislodged during stimulation and activation.
Anconeus
This muscle is examined with the patient seated or lying supine, the pronated forearm resting on the examining table or armrest ( Fig. 17-11 ). The active recording electrode is placed over the belly of the muscle, approximately three fingerbreadths distal to the olecranon, at the apex of an isosceles triangle with its base formed by the olecranon and the lateral epicondyle. It may be necessary to move the electrode slightly more anteriorly toward the lateral border of the ulna to record a monophasic CMAP. The reference electrode is placed on the posterior surface of the forearm. The radial nerve is stimulated in the lateral intermuscular septum, 1 to 2 cm proximal to the lateral epicondyle. Supramaximal stimulation is usually possible with relatively low-intensity stimulation. Activation of the muscle is achieved by the patient extending the elbow against resistance, although it may be difficult to keep the stimulating electrode in place during this maneuver. In patients with generalized myasthenia gravis, repetitive stimulation of the anconeus was found to be more sensitive than a hand muscle and equally as sensitive as the deltoid.

Deltoid
The deltoid muscle is tested with the patient seated, the active recording electrode being placed on the belly of the muscle and the reference electrode on the acromion. The arm is held adducted against the side. Activation is produced by the patient abducting the arm against resistance. Stimulation at Erb’s point is performed with a surface electrode pressed firmly behind the clavicle. With this positioning of the electrode, the patient is uncomfortable and it is not possible to avoid stimulating other muscles. Comparison studies suggest that a decremental response is found in the deltoid slightly more often than in the trapezius in myasthenia gravis.
Trapezius
The trapezius is the easiest shoulder muscle to test. The patient is examined while seated on a chair ( Fig. 17-12 ). The arm is immobilized by having the patient grip the bottom of the chair. The active recording electrode is placed on the belly of the trapezius at the angle between the neck and the shoulder, and the reference electrode is placed on the acromion. The spinal accessory nerve is stimulated at the posterior border of the sternocleidomastoid muscle where it is superficial and can be stimulated maximally with low-intensity pulses; this minimizes discomfort and avoids stimulating other muscles. Activation of this muscle is produced by the patient shrugging the shoulder while holding the bottom of the chair or while the examiner pushes down on the top of the shoulder.
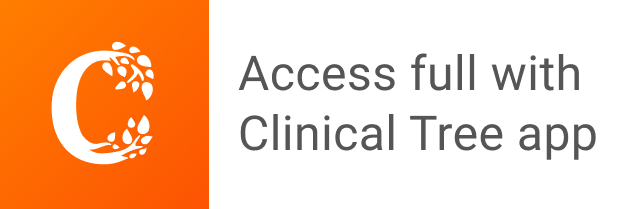