Over the last three decades, ultrasound technology has evolved such that clinicians working in small laboratories can now use it to image nerve and muscle routinely. Growing evidence has established its diagnostic utility in neuromuscular disease.
Electrophysiologic and ultrasonographic studies are complementary. Whereas electromyography (EMG) and nerve conduction studies capture electrophysiologic alterations in neuromuscular disease, ultrasound captures anatomic pathology and functional changes in terms of size, movement, and blood flow. Both electrodiagnostic and ultrasound studies involve real-time technologies that can explore limited sections of the neuromuscular system sequentially. It follows that the physician who performs the clinical and electrodiagnostic examination and who thereby knows the likely site of pathology is probably the individual best suited to conduct the ultrasound examination. This chapter will review the basic principles of neuromuscular ultrasound and the manner in which it can be used to enhance the diagnosis and care of patients.
Theoretical and technical aspects of ultrasonography
The principles of ultrasound technology are considered in detail elsewhere. The use of the oscilloscope in ultrasonography lagged behind its use in EMG because of the need to develop a suitable transducer that could send pulses of sound energy into tissue and reconvert returning echoes into electrical signals for display. It was this step that made ultrasound practical for routine diagnostic use and which eventually led to the conversion of graphic displays of time and amplitude into real-time images.
Piezoelectricity
The bulky electromagnets and diaphragms traditionally used for interconverting sound and electrical energy (e.g., in microphones and speakers) are not well suited to the study of human tissue. However, piezoelectric crystals fit perfectly the size and power requirements for ultrasound examinations. Initially, these were quartz (silicon dioxide) crystal chips that had an asymmetric molecular conformation and charge. When a small current was applied across them, it predictably would excite atoms in each molecule into different orbits, slightly altering the shape of the chip. This abrupt shift generated a brief sound pulse. Perhaps of even greater importance was the reverse property. When struck by a returning echo, the same crystal chip would respond by generating a small electrical current that correlated in its size to the intensity of the echo. Using this type of piezoelectric crystal, it became possible for a transducer to send sound energy into tissue, and then to record the latency of the returning echoes and their respective intensities.
Modern piezoelectric materials are lead zirconate ceramics that are more conducive to the shapes required to create modern multi-element, multifrequency ultrasound probes.
Display Modes
The initial ultrasound recordings involved a single crystal chip generating a single ultrasound pulse into tissue and a subsequent recording of the echoes that returned over time. These simple recordings displayed latency on the x-axis and amplitude on the y-axis, similar to a standard electrodiagnostic recording. This basic ultrasound method is termed amplitude or A-mode recording. This type of recording was capable of measuring the distance between parietal bones in a developing fetus or assessing a midline shift in a calcified pineal gland in an adult, but it was a far cry from modern ultrasound capabilities.
However, even simple A-mode recordings could be complicated by problems such as the profound loss of sound energy as it travelled through and was reflected by tissue. This meant that echoes from deeper structures were predictably much smaller in intensity than those from near structures, even if they occurred at similar types of tissue interfaces, because of the attenuation of sound energy over distance. To adjust for this, attenuation estimates of normal tissues were calculated and a correction factor applied automatically to enhance distant (later) echoes so that they would be comparable in displayed amplitude to near (sooner) echoes. This process is called time–gain compensation and is an automatic adjustment in all ultrasound instruments subject to manual override. In most instruments there is a small set of levers on the control panel that allows the operator to adjust the degree of time–gain compensation at different layers of an image ( Fig. 16-1 ).

Once the problems of generating sound, recording echoes, and adjusting for the loss of sound energy over distance in human tissues were addressed with piezoelectric transducers and time–gain compensation, useful A-mode displays could be obtained reliably and still are used in some ophthalmic circumstances. But the numerical measurements they generated proved to be of limited diagnostic capacity. Because A-mode images contained only one line of data which varied considerably with variations in the angle of incidence, ultrasound was viewed primarily as an internal caliper useful for measuring distances between a small set of distinct tissue interfaces.
It subsequently became apparent that data from multiple transducers (or one transducer moving from side to side), if coordinated reliably together, could help provide a broader assessment of more tissue. However, in a two-dimensional display, it was not possible to superimpose multiple A-mode traces to create an informative image because of the clutter involved. The next key advance in ultrasound was the development of brightness-mode (B-mode) display. This is also known as gray-scale ultrasound. B-mode display is a way of compressing an A-mode display into a single line of data that codes amplitude not as a variation on a different axis, but as brightness. The amplitude of a returning echo therefore is coded as brightness, with the strength of the brightness correlating with the intensity of the echo. When multiple lines of B-mode from an array of transducers are stitched together, it creates a single, spatial image, in the same way a television creates an image from multiple parallel lines of data that can be updated many times per second. With this display, the bright edges of tissue interfaces are visualized and tracked easily, and their changes in real time observed. A quick glance at this type of image display, one that can be checked against anatomic specimens or magnetic resonance images, provides a self-evident display of the intuitive validity and reliability of the technique.
Few currently available instruments display A-mode images. However, an image similar to A-mode can be inferred from an M-mode display. In M-mode imaging, a single line of gray-scale data is recorded, but this information is updated over time, in a display in which time is on the horizontal axis and depth on the vertical axis ( Fig. 16-2 ). This view, although not used often, is helpful for measuring temporal events, such as intervals between tremor bursts or the duration of fasciculation potentials.

Ultrasound Probes
Modern probes used in neuromuscular ultrasonography have more than 100 individual transducer elements arranged in a linear array. The long footprint of the probe is determined by the number of transducer elements and determines the number of lines of data and the width of the displayed image. Probes with smaller footprints (e.g., hockey stick design) have correspondingly smaller displays. The thick cord attached to the base of the transducer reflects the amount of wiring required for all of the individual elements. The casing of the transducer is designed to help match tissue impedance to enhance transmission of sound into tissue, and to dampen the reverberations of the piezoelectric elements and reduce the duration of the sound pulse. Given the complexity of this device, the ultrasound probe is expensive but, because it addresses the wiring needs of the patient in a single simple device, it significantly reduces the time required to complete a study.
The thickness of the transducer element determines the emitted frequency, and the instrumentation that drives the probe determines the duty factor, which is the percentage of time that the probe is emitting sound pulses. The emission of sound pulses is typically less than 1 percent of the time, with the remaining 99 percent being used to record returning echoes. (With color Doppler, the duty factor increases, leading to more tissue insonation and potential tissue heating.) The instrument controls a number of other functions of the probe, and these can be explored in greater detail in other sources.
Sound and Echoes in Tissue
The speed of sound is determined by the medium through which it passes. Harder or stiffer substances conduct sound faster than softer or looser structures, so the speed of sound in air is much slower than that in steel. This is why an approaching train is detected better by listening on the rail than through air. Most human tissues conduct sound between 1,450 m/sec (fat) and 1,585 m/sec (muscle). By convention, all ultrasound instruments base their displays on the assumption that sound travels at 1,540 m/sec, which is an average speed of sound in human soft tissues. The displayed depth of a tissue that generates an echo is based on this estimate of the speed of sound. However, because some tissues conduct sound faster or slower than this, there is a subtle but unflattering bias in that ultrasound tends to overestimate slightly the thickness of fat (by about 6 percent) and underestimate the thickness of muscle (by about 3 percent). These changes may account for subtle differences between magnetic resonance imaging (MRI) and ultrasound estimates of tissue volumes but otherwise have a negligible impact on side-to-side comparisons or serial ultrasound measurements.
The key to ultrasound lies in the tendency for a small amount of an emitted sound pulse to be reflected back from a tissue interface and for a large part of it to be transmitted through the interface to reach deeper structures. The physics of this relationship are fairly straightforward, in that the percentage of reflected sound is directly proportional to the difference in acoustic impedance of the two interfacing tissues (where acoustic impedance is related directly to the speed of sound in each tissue). As such, the subtle variation in sound speed found in human tissues is ideal for echo-based imaging, allowing for small detectable echoes at tissue interfaces and continued penetration of most sound energy to deeper structures. Problems, however, do arise with air-filled structures (e.g., lungs, 331 m/sec) and bone (4080 m/sec), which have markedly different conduction speeds than human soft tissue. For both air and bone, most sound is reflected at the interface, leaving virtually none for transmission and imaging of deeper structures. Ultrasound probes are designed with several impedance-matching layers covering the actual transducer elements and require imaging gel to couple them to the skin in order to avoid the profound loss of insonated energy that would otherwise occur between a bare transducer element and the skin.
Of course, not all sound is reflected or transmitted in tissue; some sound energy is absorbed as well. For every centimeter of depth, a certain amount of sound energy is lost (attenuation). Unlike the speed of sound in tissue, which is unrelated to the frequency of sound, attenuation is related directly to sound frequency. Higher frequencies attenuate more than lower frequencies, a property true not only in tissue but also in air. This is the reason that the low-pitched rumble of lightning as thunder is audible for miles, whereas the high-pitched and ominous crack of a lightning strike is audible only to those nearby. The degree of sound attenuation correlates with the degree of sound energy absorption and therefore with the heating of tissue. This property is of some therapeutic use, as ultrasound can be used to heat tissue. Because it is absorbed preferentially by deeper structures such as tendons, ligaments, and bones, ultrasound can deliver therapeutic heating to deep musculoskeletal structures without requiring excessive heat at the surface.
As is the case with the speed of sound in tissue, the degree of attenuation of sound in tissue is assumed to be constant by the ultrasound instrument and is corrected for automatically by a time–gain compensation algorithm. However, the degree of attenuation of sound in tissue is not constant and varies with the type of tissue and the presence or absence of pathology. Manual override of the time–gain compensation is possible on almost all machines, and this can be used to correct the image display more accurately.
Two common ultrasound artifacts result from tissues that cause either too much or too little attenuation. Calcifications in tissue, from atherosclerosis, inflammation, or trauma, reflect almost all the incident sound, casting what appears to be a shadow on the image display ( Fig. 16-3 ). Sometimes, because of directional backscattering, enhanced reflection is not readily apparent, but whenever shadowing is seen, it typically points to something that does not permit the transmission of sound, such as bone, air, or calcium. In contrast, sometimes a tissue, such as a fluid-filled cyst, causes minimal attenuation of sound. As a result, structures immediately deep to a cyst appear brighter than normal, because more sound energy is available to generate echoes. The presence of acoustic enhancement is evidence of a superficial structure that has reduced sound attenuation ( Fig. 16-4 ).


A third ultrasound artifact results from another sound reflection property of soft tissue, known as anisotropy. Some tissues backscatter reflected sound in all directions, whereas others tend to reflect insonated sound more like the way a mirror reflects light, in a defined ray or beam that forms an angle of reflection equal to the angle of incidence. The more a tissue approximates an acoustic mirror, the more it is said to have anisotropy. Tendons have high anisotropy and therefore appear very bright when the angle of incident sound is at 90 degrees to the transducer, but very dark when the probe angle changes just a few degrees. Nerves have much less anisotropy, and therefore show little difference in echogenicity when the probe angle changes from the perpendicular. Since anisotropy is tissue dependent, varying the probe angle helps distinguish different tissues from one another ( Fig. 16-5 ).

Table 16-1 summarizes the ultrasound physics discussed in this section.
Tissue-Specific Value | Transducer Frequency | Acoustic Impedance Difference at Tissue Interfaces | Ultrasound Power | Sensitivity (gain) | |
---|---|---|---|---|---|
Speed of sound in tissue | Y | U | U | U | U |
Tissue attenuation | Y | P | U | U | U |
Degree of anisotropy | Y | U | U | U | U |
Display: tissue echogenicity | Y | U | U | P | P |
Display: tissue interface echogenicity | N | U | P | P | P |
Angle of through transmission | N | U | P | U | U |
Normal and diseased muscle
Ultrasound of Normal Muscle
Normal muscle has a characteristic appearance on ultrasound ( Fig. 16-6 ). On axial images, it consists of a largely hypoechoic (dark) matrix with interspersed bright small curvilinear elements. On sagittal images, these bright intrusions are seen to be the fibrous supporting tissues of muscle, primarily epimysium and perimysium. Sometimes a prominent aponeurosis is apparent and is continuous with a muscle’s tendinous attachment. Patients can contract their muscles on request, which leads to focal bulging and movement, making muscle recognition simple. Muscle has a modest degree of anisotropy, so small adjustments in the angle of insonation of the probe change the brightness of muscle. As such, by rotating the probe to show the difference between axial and sagittal views, moving the probe distal and proximal to show the belly of the muscle versus its tendinous insertions, asking the patient to contract and relax, and by changing the angle of incidence of the probe, the identification of muscle and its distinction from other nearby tissues is relatively straightforward. During an examination, sonographers constantly move the probe and change the angle of insonation as they interrogate human tissue. These movements, and sometimes maneuvers by the patient, enable examiners to recognize tissues of interest and their relationships to nearby structures.

For clinical neurophysiologists, electrodiagnostic studies are an extension of the physical examination. In like manner, ultrasound allows for detailed exploration of specific components of the physical examination. Ultrasound provides basic information regarding the location, depth, and extent of nerve and muscle—items of particular interest in evaluating patients with dysmorphic features, anatomic variants, surgical re-attachments, or congenital abnormalities—but it also provides valuable additional information about the surrounding anatomy and systemic vascular supply to these tissues.
Reference values for the thickness of normal human muscles, adjusted for age and sex, have been published, including significant side-to-side differences. In addition, there are both qualitative and quantitative data about the echogenicity of normal human muscles, and the values vary from muscle to muscle. For example, the triceps tends to be hypoechoic and the tibialis anterior hyperechoic compared to each other (see Fig. 16-6 ).
Relatively little has been published on normal imaging of muscle contraction and relaxation. Using M-mode, the mechanical contraction of normal human muscle in response to supramaximal nerve stimulation can be recorded. This highlights the differences between the compound muscle action potential (CMAP), which is a recording of the surface membrane potential of muscle, and the actual mechanical contraction, including the phases of shortening and relaxation that follow ( Fig. 16-7 ). The kinesiology of muscle contraction can also be studied with ultrasound. For example, ultrasound can depict differences in the relative activation of vastus intermedius and rectus femoris with different leg movements ( Fig. 16-8 ).





Another aspect of ultrasound imaging that provides useful physiologic information about muscle is blood flow. Even a modest amount of exercise significantly increases muscle blood flow, as detected by power Doppler ultrasound. With ultrasound contrast agents, this increase is even more apparent. Nerve blood flow is an area of increasing interest as well. Preliminary studies suggest that blood-flow changes may provide useful information about certain types of inflammatory or compressive disorders.
Ultrasonographic Changes in Muscle Disease
The earliest reports of ultrasound in muscle disease were in muscular dystrophy, and the original observations by Heckmatt and colleagues some 30 years ago are still applicable. Muscle disease typically causes: (1) increased echogenicity of muscle; (2) loss of heterogeneity in muscle (which is the loss of ability to distinguish between muscle tissue and areas of fibrous tissue within muscle); (3) atrophy (although rarely hypertrophy is also seen); and (4) loss of bone shadow deep to muscle (acoustic shadowing). These findings are the result of disruption of the normal architecture of muscle and correlate with the degree of histopathologic change in muscle. As normal muscle fibers become necrotic or inflamed and are replaced by fat or fibrosis, more tissue interfaces that reflect sound are created. In consequence, more sound is reflected, increasing echogenicity, and more is absorbed, leading to relative acoustic shadowing of bone. The findings are seen in both myopathy and in neurogenic causes of muscle loss ( Fig. 16-9 ). Ultrasound changes are not characteristic of a specific etiology, but in general neurogenic disorders tend to cause a more moth-eaten and irregular pattern, and myopathic disorders a more diffuse, homogeneous change (a generalized smudge). More helpful, often, is the distribution of ultrasound findings. For example, in inclusion-body myositis the changes are most pronounced in forearm flexors and quadriceps, whereas in generalized neuropathies abnormalities are conspicuous in the most distal muscles. A few rare disorders have characteristic findings, such as Bethlehem myopathy, in which there is a characteristic “outside-in” pattern.
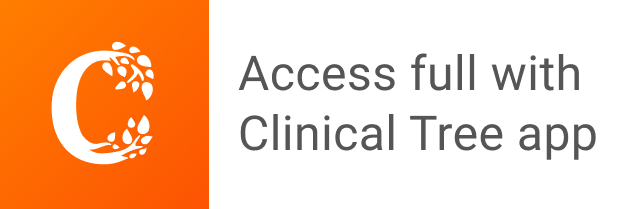