Averaged evoked potentials have been used widely to record the changes in electrical potential that occur within the nervous system in response to an external stimulus. For clinical purposes, the short-latency brainstem auditory evoked potential (BAEP), somatosensory evoked potential (SEP), and visual evoked potential (VEP) are recorded. In general, these evoked potentials represent an obligate neuronal response to a given stimulus, and both their amplitude and latency depend on the physical characteristics of the eliciting stimulus (see Chapter 22, Chapter 23, Chapter 24, Chapter 25, Chapter 26, Chapter 27 ). Such “exogenous” or “stimulus-related” potentials (SRPs) are independent of whether the subject is attentive to or interested in the stimulus. Indeed, BAEPs, SEPs, and flash VEPs can be recorded even when the subject is asleep.
There is, however, another distinct class of evoked potential: the “endogenous” or “event-related” potentials (ERPs) that can be recorded in response to an external stimulus or event. These potential changes, unlike the SRPs just described, occur only when the subject is selectively attentive to the stimulus and are elicited only in circumstances in which the subject is required to distinguish one stimulus (the target) from a group of other stimuli (the nontargets). They depend primarily on the setting in which the target stimulus occurs and are relatively independent of the physical characteristics of that stimulus. Thus, ERPs seem to be related to some aspect of the cognitive events associated with the distinction of target from nontarget stimuli. Attempts have even been made to relate ERPs to presumed stages of information processing (e.g., sensory discrimination or response selection) that must be completed successfully before a person can respond selectively to a target stimulus. The precise relationship between any such stages and the individual components of the ERP is, however, uncertain. Indeed, it seems most likely that the ERP reflects activity in distributed parallel networks that are responsible for the discriminative behavior.
Because of this relationship between ERPs and cognitive behavior, considerable interest has developed in the possible clinical use of these potentials in the evaluation of patients who suffer from disorders of cognition. This interest has focused principally on disorders that affect brain function diffusely (e.g., Alzheimer disease, frontotemporal dementia, schizophrenia, or metabolic encephalopathies).
Description of event-related (endogenous) potentials
Several components of the ERP have been identified; these include Nd ; the processing negativity (PN ); the mismatch negativity (MMN ); P165 ; N2 ; P3a ; P3 (or P3b ); N400 ; and the error negativity (ERN or N e ). With the exception of P3 (which is also referred to as the P300 component because of its polarity and latency), these components have not been found consistently in different recording situations. Much of this apparent inconsistency probably relates to two factors. First, these other ERP components are of relatively small amplitude compared with the P3 component and thus are more difficult to separate from background noise when only a few trials are averaged. Second, most of these components occur at relatively short latencies and overlap considerably with the simultaneously occurring SRPs. As a consequence, special procedures such as the subtraction of one waveform from another are often required for demonstrating them ( Fig. 29-1 ). Most clinical studies therefore have been concerned primarily with the more easily identified N1 and P2 components in response to the frequent tone and the P3 component in response to the rare tone (see Fig. 29-1 ). For this reason, attention is confined to a discussion of these components of the ERP in the remainder of this chapter.

The simplest experimental design that is used to elicit the P3 response is the so-called oddball paradigm. In this design, the subject is presented with a sequence of two distinguishable stimuli, one of which occurs frequently (the frequent stimulus) and the other infrequently (the rare stimulus). The subject is required to count mentally or otherwise respond to one of the two stimuli. Cerebral responses to the rare and frequent stimuli are recorded and averaged separately.
The response to the frequent stimulus consists of a series of waves (the stimulus-related components) that relates, for the most part, to the sensory modality stimulated. For example, to an auditory stimulus this response has been divided into three sequential time periods: (1) early-latency, (2) mid-latency, and (3) long-latency responses ( Fig. 29-2 ). The early-latency (less than 10 msec) response (BAEP) reflects activity in the peripheral and brainstem auditory structures (see Chapter 24 ). The mid-latency (10 to 50 msec) response is thought to reflect a combination of muscle reflex activity and neural activity that may arise in the thalamocortical radiations, primary auditory cortex, and early association cortex. The neural generators of the long-latency (greater than 50 msec) response are uncertain, although probably the ERP reflects overlapping neural activity from multiple neocortical and limbic regions. This response consists of a large negative (N1)–positive (P2) complex referred to as the “vertex potential” because it has the largest amplitude at the vertex. At least part of this vertex potential seems to reflect activity in neural areas that can be activated by more than one sensory modality. Thus, a similar negative–positive complex is elicited by auditory, visual, and somatosensory stimuli ( Fig. 29-3 ). This response is nevertheless an obligate response of the nervous system to the stimulus and is largely independent of the subject’s attention or level of arousal. It is, therefore, like the early-latency and mid-latency components, a stimulus-related response.


In contrast, the long-latency response to the rare auditory stimulus is considerably different and consists of a negative (N1)–positive (apparent P2)–negative (N2)–positive (P3) complex (see Fig. 29-1 ). The first positive wave is termed apparent P2 because it represents the sum of the stimulus-related P2 and the event-related P165. This response is quite consistent, in both amplitude and latency, in the same subject performing the same task even when measured on several different occasions over a period of months ( Fig. 29-4 ). The P3 component of this response has a latency-to-peak of approximately 300 to 400 msec following onset of the rare stimulus; it is of positive polarity and is of maximal amplitude in the midline over the central and parietal regions of the scalp. An evoked potential component with a similar scalp distribution can be recorded to stimuli in any of the sensory modalities (see Fig. 29-3 ) and can even be recorded (without associated SRPs) when an anticipated stimulus is omitted unexpectedly. The neural generators of this P3 response are not known definitively, although converging evidence has suggested a temporo-parietal source.

Several variables alter the amplitude and latency of the SRPs without appreciably affecting the ERPs, and vice versa. For instance, a change in the intensity of stimulation has relatively little effect on the P3 component ( Fig. 29-5 ) but has a major influence on the associated SRPs (see Chapter 22 , Chapter 23 , Chapter 24 , Chapter 25 , Chapter 26 , Chapter 27 ). Conversely, the P3 is influenced by changes in the ease with which targets can be distinguished from nontargets ( Fig. 29-6 ), by alterations in the ratio of target to nontarget stimuli ( Fig. 29-7 ), or by shifts in the attention of the subject (see Fig. 29-1 ), whereas SRPs are not.



Recording arrangements
To record ERPs it is necessary to be able to deliver both frequent and rare stimuli and to average separately the cerebral response to each. Any sensory modality can be used, although in clinical practice an auditory stimulus is most common. The stimuli are generally two or three differently pitched tones (see Fig. 29-1 ) delivered binaurally with a relatively long interstimulus interval (i.e., greater than 1 second) because of the long refractory period of the vertex potential.
The amplitude of the P3 response is quite large (i.e., 50 to 100 times the amplitude of the BAEP), and therefore an average of the cerebral response to a relatively low number of rare tones is generally sufficient to improve the signal-to-noise ratio to the point that the P3 response can be easily seen ( Fig. 29-8 ). Indeed, the P3 amplitude is often so large that the P3 response can be identified in single trials (see Fig. 29-8 ). The probability of rare tone occurrence generally is set between 10 and 30 percent, so that approximately 50 responses to the rare tone can be averaged in 10 minutes of recording time. This arrangement seems to be a good balance between the enhancement of P3 amplitude that occurs at smaller ratios of target to nontarget stimuli, the increased response noise associated with fewer trials in the rare tone average, and the difficulty of keeping the subject’s attention for longer recording times.

Optimally, multiple recording channels (a minimum of four) are used. Responses are recorded from Fz, Cz, and Pz electrode placements on the scalp referenced to an indifferent (although not necessarily inactive) scalp location such as the mastoids or ear lobe. Eye movements may contaminate the ERP recordings; therefore, at least one channel usually is devoted to monitoring them. Depending on the recording apparatus used, it may be possible to reject automatically trials containing eye movement or to remove digitally eye blink artifacts from the responses and thereby produce less noisy recordings.
The electrical power in these long-latency responses essentially is confined to frequencies under 10 Hz so that the high-frequency cut-off of the filters used (down 3 dB at the cut-off frequencies; roll-off of 12 dB per octave) can be set low enough (e.g., at 40 to 50 Hz) to eliminate any 60-Hz activity that otherwise might degrade the waveforms. The best low-frequency cut-off is somewhat controversial, although it probably should not exceed 1 Hz because much of the power in these recordings is in the 1- to 4-Hz frequency band, and a marked distortion in P3 amplitude begins to occur at this point. In addition, as will occur with any analog lowpass filter, responses will be shifted to earlier latencies at even very low filter settings, so it is important to record both normal control subjects and clinical patients at the same bandpass.
Non-neurologic factors influencing the P3 component
Several non-neurologic factors may affect the ERP; it is essential that these factors be taken into account if ERPs are to be recorded in a clinical setting.
Age
Advancing age, during both maturation and senescence, has an important influence on several of the long-latency evoked potential components ( Fig. 29-9 and Table 29-1 ). The data on maturation are limited, but they suggest that the stimulus-related N1 and P2 components have reached their adult latencies at least by the age of 5 or 6 years and perhaps well before. The event-related N2 and P3 components, in contrast, are markedly prolonged in young children and progressively decrease in latency with increasing age until reaching adult values in the teenage years or early twenties. This apparently differential effect of maturation on the stimulus-related and event-related components may be related to the temporal evolution of cognitive development in children.

Slope | Correlation Coefficient | Standard Error about Regression Line | Value at Age 15 Yr | Significance | |
---|---|---|---|---|---|
Stimulus-Related Components | |||||
N1 latency | 0.1 msec/yr | 0.228 | 8 msec | 94 msec | NS |
P2 latency | 0.7 msec/yr | 0.560 | 19 msec | 168 msec | P < .001 |
N1–P2 amplitude | −0.2 μV/yr | −0.420 | 5.56 μV | 15.6 μV | P < .01 |
Event-Related Components | |||||
N2 latency | 0.8 msec/yr | 0.691 | 15 msec | 199 msec | P < .001 |
P3 latency | 1.6 msec/yr | 0.810 | 21 msec | 310 msec | P < .001 |
N2–P3 amplitude | −0.2 μV/yr | −0.313 | 7.9 μV | 17.7 μV | P < .05 |
Beginning in about the midteens, a linear increase occurs in the latency of both the stimulus-related (P2) and the event-related (N2 and P3) components with advancing age (see Fig. 29-9 and Table 29-1 ). Several studies have corroborated these general findings ( Table 29-2 ), although the rate of these changes has varied somewhat between reports. Although occasional reports have suggested that the age-related change in the latency of the P3 component is curvilinear, most authors have found the P3 latency–age function to be linear. For example, in a meta-analysis of the existing literature on this issue as of 1996, Polich concluded that the weight of the evidence supported a linear, and not a curvilinear, relationship between the latency of P3 and age. This has continued to be the experience of others. As a result of these discrepancies, it is unclear whether, or in what circumstances, nonlinear factors are important determinants of the P3 latency–age function. For example, even in the study of Anderer and associates, the significance of such curvilinear effects may have been exaggerated by the fact that the four oldest subjects (greater than 80 years) had quite deviant P3 latencies. It would be of interest to know what effect the exclusion of these four subjects from the analysis may have on the significance of the curvilinear nature of the age–latency function. In addition, the results of one study, in which a high level of cognitive function was strictly controlled, indicated that there were no significant age-related changes in either P3 latency or amplitude. Although this study has been criticized, the findings are provocative and clearly worth replication. Regardless, however, even based on this study, when using a specific testing procedure on individuals of uncertain status, a comparison with age-adjusted controls from an unaffected population is appropriate.
Latency | Amplitude | |||||
---|---|---|---|---|---|---|
Slope (msec/yr) | Intercept (msec) | SE (msec) | Slope (μV/yr) | Intercept (μV) | SE (μV) | |
Goodin et al * | +1.64 | 285 | 21 | −0.18 | 17.6 | 5.56 |
Picton et al * † | +1.71 | 294 | 25 | −0.15 | 16.6 | 4.0 |
Brown et al * | +1.12 | 272 | ‡ | −0.15 | ‡ | ‡ |
Pfefferbaum et al * | +0.94 | ‡ | 51 | −0.13 | ‡ | ‡ |
Emmerson et al * | +1.46 | ‡ | ‡ | ‡ | ‡ | ‡ |
Gordon et al * | +0.91 | ‡ | 31 | ‡ | ‡ | ‡ |
Enoki et al | +1.43 | 297 | 21 | ‡ | ‡ | ‡ |
Iragui et al | +0.88 | 294 | 44 | −0.10 | 14.4 | 5.1 |
Polich | +0.92 | 304 | 32 | ‡ | ‡ | ‡ |
Anderer et al | +0.92 | 333 | 33 | −0.15 | 22.4 | 5.4 |
Alain and Woods | +1.4 | ‡ | ‡ | −0.25 | ‡ | ‡ |
Walhovd and Fjell | +1.65 | 278 | ‡ | −0.17 | 19.3 | ‡ |
Schiff et al | +1.7 | 270 | 30 | −0.04 | 19.3 | 2.1 |
† Data reported for auditory stimulation with an interstimulus interval of 3.3 seconds and a 30 percent probability of rare tone occurrence.
This age-related increase in latency occurs at a faster rate for components of longer initial peak latency, which suggests that a relatively uniform slowing of neural transmission occurs with advancing age. The intersubject variability in P3 latency is generally small in relation to the actual peak latency, and most authors report standard errors around the regression line of between 20 and 30 msec (see Table 29-2 ).
With increasing age, the amplitudes of both the stimulus-related and the event-related components of the long-latency evoked potential also decrease (see Table 29-1 ). The intersubject variability in amplitude is, however, extremely large, so a single standard error around the normal regression line is equivalent to approximately 60 to 80 percent of the expected P3 amplitude at 60 years of age (see Table 29-2 ). Such marked variability limits the usefulness of amplitude measurements in a clinical setting.
Sex
Gender is known to have effects on several of the stimulus-related components used clinically (see Chapter 22 , Chapter 23 , Chapter 24 , Chapter 25 , Chapter 26 , Chapter 27 ). By contrast, this factor does not seem to have any effect on latency of the event-related components, although the amplitude of P3 may be larger in female subjects.
Drugs
An understanding of the changes in the ERP produced by drugs (in particular, antipsychotics and antidepressants) is extremely important. Certainly, drugs taken in dosages sufficient to produce a metabolic encephalopathy can affect the ERP, but the effect of these particular drugs taken in therapeutic dosages seems to be minimal. Thus, an evidence-based review concluded that, while an amplitude reduction was possibly helpful diagnostically, medication status was not a factor. Similarly, Ford and co-workers studied 21 unmedicated schizophrenic patients. The subjects were tested after receiving placebo for 1 week and again after treatment with antipsychotic medication for 4 weeks. Despite significant clinical improvement while taking medication, the amplitude and latency of the N1, N2, and P3 components of the response were unchanged. Consequently, it seems that these medications have relatively minor effects on ERPs in therapeutic dosages.
Several researchers have looked at the effect of other medications on the ERP. Particular interest has been directed at drugs that influence the cholinergic system, and several authors have reported a significant increase in the amplitude and shortening in the latency of the P3 response in patients with Alzheimer disease treated with cholinergic medication. Moreover, an opposite effect has been observed with the use of anticholinergic medications or with thiamine. These changes cannot, however, be interpreted as a specific effect on the generators of the P3 response because P3 amplitude changes may result from a general change in the attention or arousal of the subject, and latency shifts may result from delays at earlier stages of processing.
The effects of anticonvulsant medications on the ERP are less clear-cut. Meador and colleagues studied, in a double-blind crossover trial, the effects of different anticonvulsants on the P3 response in patients with epilepsy. They found no difference in P3 latency or amplitude between patients taking carbamazepine, phenobarbital, or phenytoin in therapeutic doses. Chen and co-workers studied the P3 response in 73 children with newly diagnosed epilepsy, both before and after 6 and 12 months of treatment with antiepileptic medication. They reported that P3 latency was unchanged in the patients receiving valproic acid and carbamazepine but was increased in patients receiving phenobarbital. By contrast, Panagopoulos and colleagues reported that treatment with valproic acid, but not carbamazepine, resulted in prolongation of P3 latency. The reason for such discrepant findings is unclear but may relate, in part, to the fact that different blood levels of the anticonvulsants, particularly in toxic ranges, may affect P3 latency.
Sleep Deprivation
Sleep deprivation has been reported to cause both an increase in the latency and a decrease in the amplitude of the P3 response, perhaps reflecting a decrease in the subject’s level of vigilance in the sleep-deprived state.
Fitness
Fitness, as determined by increased oxygen utilization during maximal exercise, has been reported to reduce P3 latency in older subjects. In one report, however, the significance of the change was only marginal (P < .05), and the finding that increased fitness in younger subjects tended to increase P3 latency makes the conclusion questionable. More importantly, in a recent study comparing ERPs in fit subjects with those in sedentary subjects, no difference was found in P3 latency or amplitude between groups. Interestingly, however, following exercise, the P3 amplitude increased and its latency decreased significantly in both groups.
Other Factors
It has been suggested that certain other variables (e.g., the recency of food consumption, body temperature, time of day, season, and position in the menstrual cycle) may affect the P3 response. These reports still need to be confirmed, but even if the findings are corroborated, the small magnitude of the reported changes makes it unlikely that these variables will markedly influence the interpretation of clinical studies.
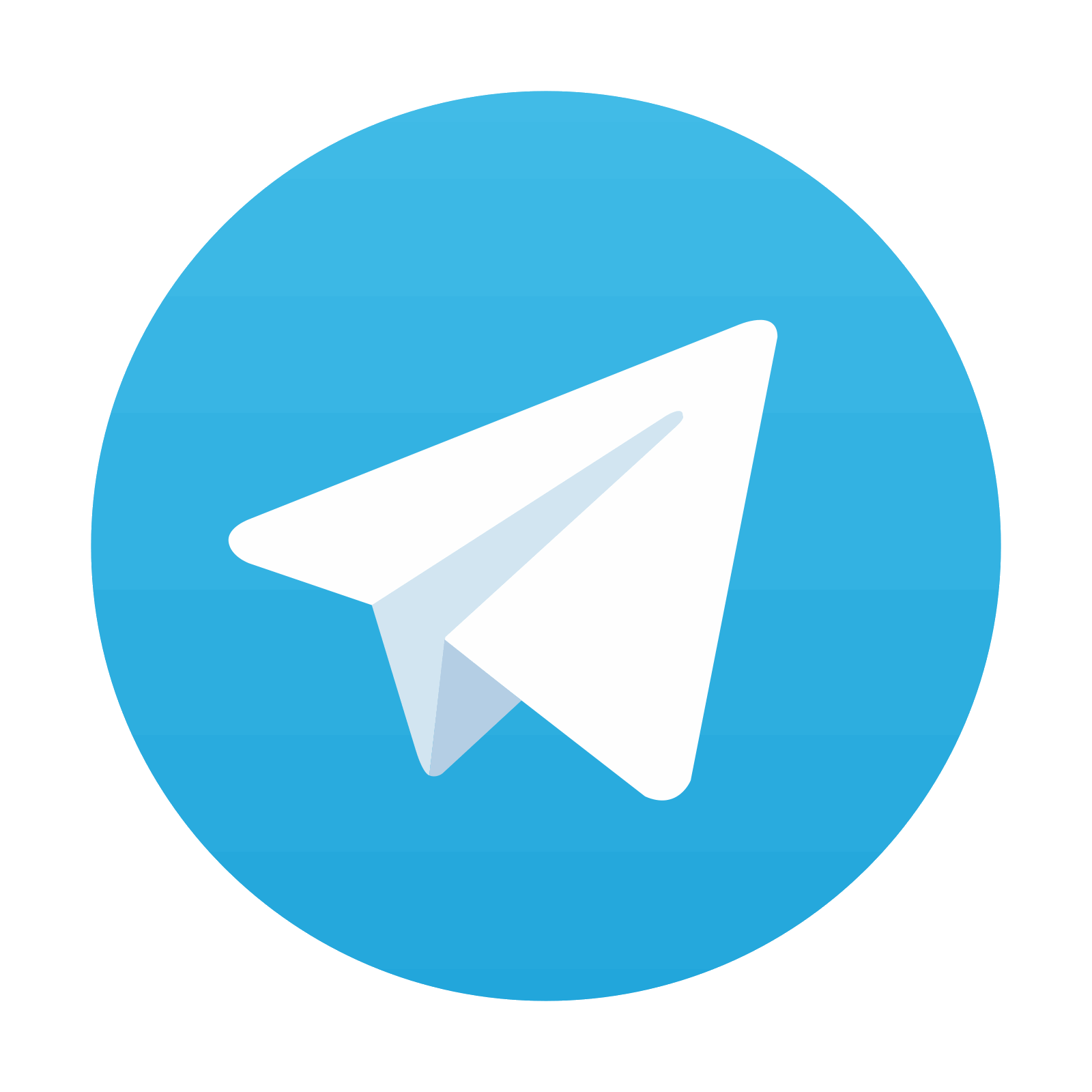
Stay updated, free articles. Join our Telegram channel
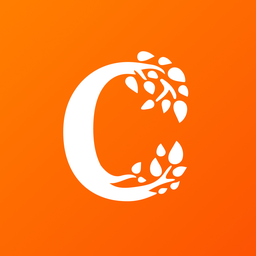
Full access? Get Clinical Tree
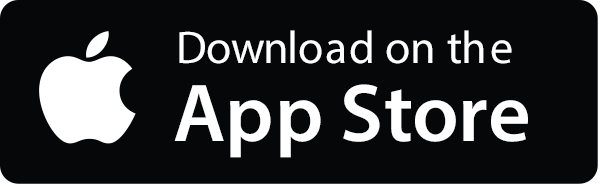
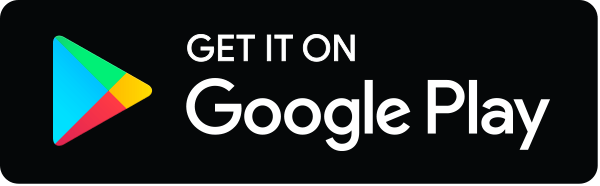