(1)
Department of Biomedical and Clinical Sciences Luigi Sacco, University of Milan, Milan, Italy
(2)
Coma Science Group, Cyclotron Research Center and Neurology Department, University and University Hospital of Liège, Liège, Belgium
Abstract
Although assessing a subject’s capacity for consciousness is commonly based on an input-output paradigm, clinical and physiological evidence advocate the development of brain-based metrics that are independent on both sensory inputs and motor outputs. As an attempt in this direction, we devised an empirical measure of complexity derived from the electroencephalographic (EEG) response to a direct cortical perturbation with transcranial magnetic stimulation (TMS), the perturbational complexity index (PCI). Based on theoretical postulates, PCI gauges the conjoint presence of integration and information in the human brain, independently of sensory inputs and motor outputs. In a preliminary series of experiments, PCI was tested on TMS-evoked potentials recorded in healthy subjects during wakefulness, dreaming, NREM sleep, and different levels of sedation induced by different anesthetic agents, as well as in patients who emerged from coma and attained a stable diagnosis. These experiments show that PCI allows a reliable assessment of the level of consciousness at the single-subject level and prompt further validation toward the development of a diagnostic test. In parallel, elucidating the mechanisms by which brain complexity collapses and recovers following brain injury may provide novel insight on the physiopathology and the treatment of disorders of consciousness.
Keywords
ConsciousnessNeurophysiologyPerturbational complexity indexTMS8.1 The Problem of Disconnected Consciousness
In everyday life and in the clinical practice, assessing consciousness relies ultimately on a behavioral input-output paradigm; we stimulate subjects in different sensory modalities and we observe their motor responses. If an individual is able to connect to the surrounding environment and then produces appropriate responses, she/he is considered conscious. Thanks to recent conceptual and technical advances, a similar input-output paradigm can now be applied even to patients who are fully paralyzed and cannot engage in any behavioral output, such as patients affected by a total locked-in syndrome [1–3]. In this case, subjects are still presented with sensory inputs, but the blockage of motor output is bypassed by recording directly neuronal activations by means of functional neuroimaging (fMRI) and electroencephalography (EEG). In this way, it is possible to detect specific neuronal markers of mental imagery being covertly performed in response to sensory stimuli and commands, thus demonstrating that some patients are aware despite their lack of behavioral responsiveness. These neuroimaging paradigms leave no doubts in case of a positive result: if the neuronal output is consistent and specific with respect to the sensory input, the subject is conscious. However, the neuroimaging approach lacks sensitivity and many conscious subjects fail to produce the appropriate neuronal responses to stimulations and commands [4].
A fundamental problem is that conscious experience can also be present in subjects who are disconnected from the environment on the input side. For example, during dreaming complex, temporally unfolding hallucinatory episodes can be as intense and vivid as waking consciousness—yet sensory stimuli are ignored to the point that they are rarely incorporated in the experience [5]. Consciousness may completely disconnect from the external environment also during some forms of anesthesia. Some dissociative anesthetic agents, such as ketamine at high doses, are known to induce a dreamlike hallucinatory state associated with sensory disconnection and complete unresponsiveness. Similar disconnections may occur in pathological conditions whereby a brain-injured subject may not respond to verbal commands or sensory stimuli because a peripheral or central lesion prevents sensory inputs from being transmitted and processed effectively. In all these cases, detecting consciousness based on an input-output paradigm may result in a significant rate of false negatives.
Clearly, finding a way to detect consciousness independent of both sensory inputs and motor outputs is a daunting proposition. One should first postulate which neural mechanisms are fundamental for consciousness, then should devise a practical way to gauge these mechanisms, and, finally, should validate this novel metric across several physiological, pharmacological, and pathological conditions in a large cohort of subjects who are able to provide an immediate (or retrospective) report about their level of consciousness. This validation should start from typical conditions in which consciousness is present (wakefulness) or lost (deep NREM sleep, seizures, anesthesia) and then extend to more ambiguous cases in which consciousness may be present but disconnected (dreaming, ketamine anesthesia). To the extent that this long process yields an index that is highly specific and highly sensitive for the presence of an immediate, or retrospective, conscious report across all these conditions, it may be possible to obtain a reliable frame of reference to detect a capacity for consciousness in patients who are completely disconnected from the external environment. Below, we describe a preliminary attempt in this direction.
8.2 Consciousness and Brain Complexity
The first step requires formulating a postulate. A parsimonious approach is to start from self-evident axioms in order to establish what physical properties are fundamental for consciousness and how they can be measured. Naturally, in the case of consciousness, evidence can only be gathered from phenomenology, the first-person observation of subjective experience itself [6]. Phenomenologically, each conscious experience is both differentiated—that is, it has many specific features that distinguish it from a large repertoire of other experiences—and integrated—that is, it cannot be divided into independent components. Neurophysiologically, these fundamental properties of subjective experience rely on the ability of multiple, functionally specialized modules of the thalamocortical system to interact rapidly and effectively to form an integrated whole [7–10]. Hence, the fundamental postulate is that consciousness requires an optimal balance between functional integration and functional differentiation in thalamocortical networks—otherwise defined as brain complexity [11–14]. This notion implies that the complexity of brain activity should be high when consciousness is present and low whenever consciousness is lost in sleep, anesthesia, or coma [12, 15, 16].
8.3 Assessing Brain Complexity Through Perturbations
The second problem entails devising an empirical measurement that must be, at the same time, practical and in line with the fundamental postulate. On the one hand, theoretical measures that have been designed to assess the joint presence of differentiation and integration in neural systems [17–19] are only applicable to simple systems of simulated elements or under highly restrictive assumptions and have not been tested on human brains. On the other hand, currently used empirical indices of consciousness are either based on integration alone [as judged by the extent or synchronization of cortical activations [20–22]] or on differentiation alone [as judged by entropy or spectral content [23, 24]] and do not reliably assess consciousness in individual patients or across many different conditions.
A viable and principled way to gauge the conjoint presence of integration and information in real brains involves directly probing the cerebral cortex (in order to avoid possible subcortical filtering and gating) by employing a perturbational approach (thus testing causal interactions rather than temporal correlations) and examining to what extent cortical regions can interact as a whole (integration) to produce differentiated responses (information) [25]. Practically, this approach can be applied to the human brain by employing a combination of transcranial magnetic stimulation (TMS) and high-density EEG, a technique that allows stimulating directly a subset of cortical neurons and measuring, with good spatial-temporal resolution, the effects produced by this perturbation on the rest of the thalamocortical system [26]. According to this proposal, a signature of consciousness is that the thalamocortical system should respond to TMS with complex, rapidly changing activity patterns (information) that affect a distributed set of cortical areas (integration). On the other hand, it can be predicted that during loss of consciousness, whether this is caused by sleep, anesthesia, or coma, the brain should react to perturbations with a response that is local (loss of integration) and/or stereotypical (loss of information) (Fig. 8.1a). In order to quantify the spatiotemporal complexity of TMS-evoked cortical activations, a novel empirical measure called the perturbational complexity index (PCI) has been recently introduced [27]. Calculating PCI involves two fundamental steps: (1) perturbing the cortex with TMS to engage distributed interactions in the brain (integration) and (2) “zipping” (i.e., compressing) the resulting electrocortical responses to measure their algorithmic complexity (information) (Fig. 8.1b). The underlying idea is that PCI should be low if causal interaction among cortical areas is reduced (loss of integration), because the matrix of activation engaged by TMS is spatially restricted; PCI is also expected to be low if many interacting areas react to the perturbation but they do so in a stereotypical way (loss of differentiation) because, in this case, the resulting matrix is large but redundant and can be effectively compressed. In fact, PCI should reach high values only if the initial perturbation is transmitted to a large set of integrated areas that react in a differentiated way, giving rise to a spatiotemporal pattern of deterministic activation that cannot be easily reduced.
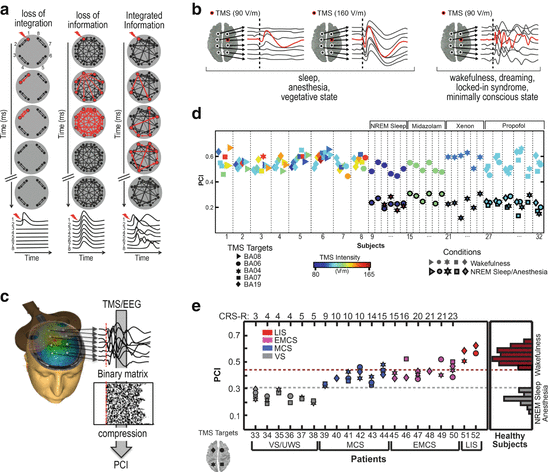
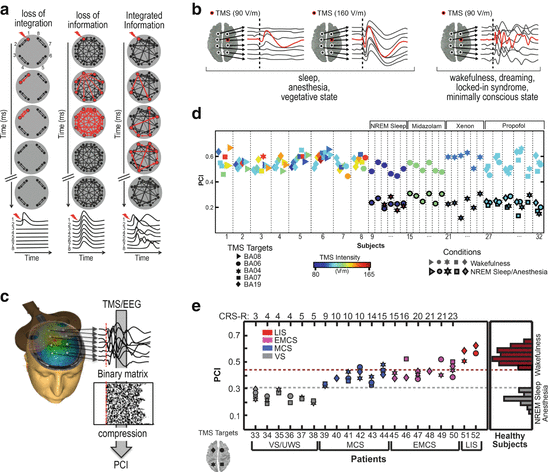
Fig. 8.1
TMS/EEG allows to test the IIT in humans and PCI quantifies the spatiotemporal pattern of deterministic brain response to perturbation. (a) Outline of the possible responses to perturbation of an idealized system. When the system is not integrated (left), the response is short-leaved and limited to locally connected elements; when the system is fully connected in a uniform way (middle), the response spreads in all the elements at the same time and then rapidly vanishes; when the system realizes an optimal balance between information and integration, the response is long-lasting and widespread, yet complex, involving different elements at different times (right). (b) Examples of the typical TMS-evoked EEG responses obtained when consciousness is lost and present. (c) PCI is computed by compressing the binary matrix of significant spatiotemporal activations evoked by TMS at the source level estimated from scalp recordings. (d, e) PCI distributions in healthy subjects during wakefulness and conditions of loss of consciousness and in brain-injured vegetative state, minimally conscious state and emergence from MCS patients
8.4 Testing the Perturbational Complexity Index in Sleep Anesthesia and Coma
In a preliminary series of experiments, PCI was tested on a large dataset of TMS-evoked potentials recorded in healthy subjects during wakefulness, dreaming, NREM sleep, and different levels of sedation induced by different anesthetic agents (midazolam, xenon, and propofol), as well as in patients who emerged from coma and attained a stable diagnosis as assessed through repeated measurements with the Coma Recovery Scale-Revised [28] (overall, 208 sessions in 52 subjects). In healthy, awake subjects, TMS triggered a complex EEG response involving different cortical areas at different times (Fig. 8.1c) [29, 30]. When subjects lost consciousness during NREM sleep, TMS pulses invariably produced a simple wave of activation that remained localized to the site of stimulation, indicating a breakdown of communication and a loss of integration within thalamocortical networks (Fig. 8.1c) [29]. The disappearance of a long-range, differentiated pattern of cortical activation was not simply due to a reduction of responsiveness of hyperpolarized cortical neurons. In fact, increasing TMS intensity only resulted in a larger, simple positive-negative wave, closely resembling a spontaneous sleep slow wave [31]. In this case, the response to TMS was stereotypical and spread like an oil spot to vast regions of the cortex, revealing a loss of differentiation (Fig. 8.1c). Similar local and/or stereotypical responses were invariably also found during general anesthesia [32] as well as in brain-injured patients with an unambiguous clinical diagnosis of a vegetative state [33] (Fig. 8.1c). Crucially, wakefulness-like, complex responses always recovered during REM sleep [34] in minimally conscious patients, who showed signs of nonreflexive activity, and in locked-in syndrome (LIS) subjects, who were totally paralyzed except for vertical eye movements through which they signal that they were aware [33].
Accordingly, in healthy (awake, sleeping, and anesthetized) subjects—whose level of consciousness can be known based on subjective reports upon awakening— PCI provided a reproducible scale along the unconsciousness-consciousness spectrum (Fig. 8.1d). Notably, brain-injured patients (MCS, EMCS, and LIS) who showed minimal signs of consciousness attained values of brain complexity that were invariably above the maximum value obtained in unconscious, anesthetized, or sleeping, healthy subjects (Fig. 8.1e).
8.5 Toward the Development of a Reliable Diagnostic Tool
The preliminary results obtained so far are encouraging but further testing will be needed before PCI can be practically employed at the bedside of disorders of consciousness (DOC) patients. First, the index needs to be validated in a larger cohort of healthy subjects, who can provide a report about their level of consciousness. This cohort should include conscious subjects affected by brain lesions with different extents and etiologies (stroke, traumatic injury, postanoxic lesions, late-stage Alzheimer disease). This testing will provide a thorough assessment of the sensitivity of PCI as well as indications on how to optimally perturb the brain with TMS in the presence of focal cortical damage or diffuse alterations. As already mentioned, PCI measurements should also be extended to conditions in which subjects are temporarily disconnected but report conscious experience retrospectively. This will involve experiments during dreaming (both in REM and NREM sleep) as well as during ketamine anesthesia. Overall, the values of PCI obtained in well-controlled conditions in which consciousness is known to be present (report, or delayed report) or absent (no report) will then be used to construct a receiver operator characteristic (ROC) analysis and to derive a cutoff that maximizes accuracy of distinction between these two conditions (Youden’s index). It will then be crucial to verify whether this cutoff confirms the sensitivity of PCI in a large cohort of minimally conscious state (MCS) patients, as identified by the CRS-R. This is a key test, since current brain-derived measures of consciousness have a high rate of false negatives in this category of patients. At this point, a diagnostic test with an established accuracy will be available to assess consciousness independently of sensory inputs and motor outputs. This diagnostic measure could be finally applied to unresponsive wakefulness syndrome (UWS) patients, whose level of consciousness is unknown. For example, finding a PCI value well above the cutoff derived from the report/no report distributions in one of these patients would suggest that she/he is conscious to some extent, albeit completely disconnected from the external environment.
8.6 Exploring the Neurophysiological Mechanisms Underlying Loss and Recovery of Consciousness
Establishing a link between consciousness and brain complexity—in both theory and practice—may also help shedding new light on the cortical mechanisms of loss and recovery of consciousness in brain-injured patients. To this aim, once again, the contrast between wakefulness and sleep offers an interesting starting point. In fact, as previously discussed, TMS-evoked EEG responses obtained in stable chronic VS/UWS patients show a remarkable similarity with those obtained during NREM sleep, thus pointing to a common electrocortical event in both these conditions.
As already described above (Fig. 8.1c), during NREM, the differentiated pattern of deterministic interactions that propagate through a distributed network of cortical areas triggered by TMS during wakefulness is replaced by a simple response that remains either local [29] or spreads like an oil spot, often matching the EEG criteria for a sleep slow wave, or a K-complex [31]. These EEG graphoelements are typically occurring during natural sleep and are associated with brief periods of neuronal membrane hyperpolarization and silence (the so-called down state) [35] known to be due to neuronal bistability. Bistability, in turn, is caused by an increased activity of leak K+ channels brought about by decreased brainstem cholinergic activity and defined as the tendency of cortical neurons to fall into a silent down state in response to transient increases in activity [36, 37].
We hypothesized that bistability may drastically impair the emergence of complex patterns of causal interactions among cortical areas. Specifically, we hypothesize that (i) during NREM a group of neurons that receives a cortical input rapidly plunges into a down state and that (ii) this period of silence breaks off the causal effects of the initial input.
In a recent study [38], we tested this hypothesis by using intracranial single-pulse electrical stimulation (SPES) and simultaneous stereotactic EEG (SEEG) recordings in 8 patients undergoing neurosurgical evaluation for intractable epilepsy during both wakefulness and NREM sleep. Intracranial recordings allow a reliable, although indirect, detection of cortical down states in terms of significant suppression of high-frequency power above 20 Hz in the LFP [39, 40]. Also, intracranial perturbations with SPES allow assessing cortico-cortical and/or cortico-subcorticocortical interactions from a causal perspective [41].
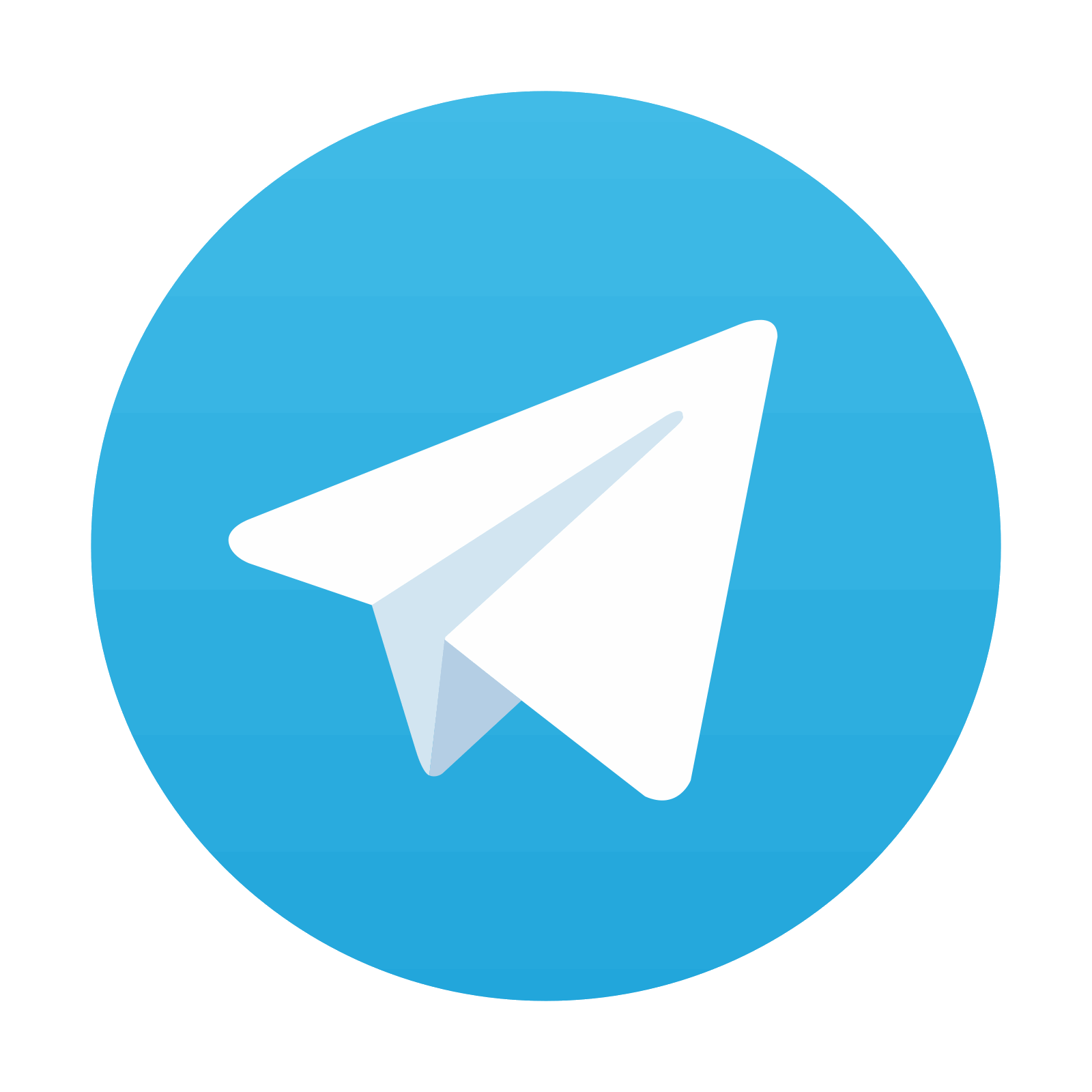
Stay updated, free articles. Join our Telegram channel
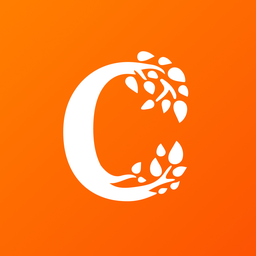
Full access? Get Clinical Tree
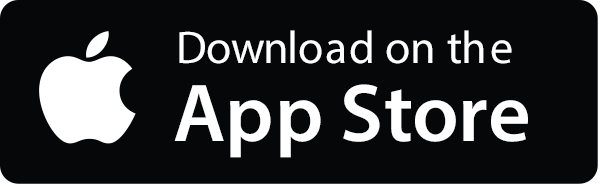
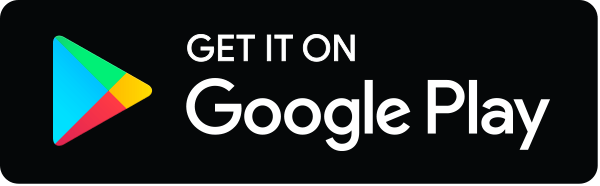