Fig. 9.1
Neurogenesis in the SVZ. (a) Schematic brain sagittal section showing the two main neurogenic niches in the postnatal brain, the dentate gyrus of the hippocampus and the SVZ (orange). SVZ-derived migrating progenitors (light blue) move tangentially along the RMS towards the OB, where they migrate radially and differentiate into inhibitory interneurons. (b) Anatomy of the SVZ. Ependymal ciliated cells (purple) line the wall of the lateral ventricle, and are in contact with quiescent stem cells (“B” cells, yellow), which in turn give rise to transit amplifying cells (“C” cells, orange). The latter produce migratory neuroblasts (“A” cells, light blue). The SVZ niche is rich in blood vessels (red). (c) (Left panel) Migratory neuroblasts slide along each other in chains surrounded by an astrocytic network. (Right panel) Typical RMS migratory neuroblasts extend a long protrusion oriented towards the OB, as shown by GFP-labeling via in vivo postnatal electroporation. The OB is located to the right of the image. (d) (Left panel) Following radial migration in the OB, neuroblasts (light blue) differentiate into either granular cells (purple) making dendrodendritic synapses with mitral cells in the GCL (green) or into periglomerular cells (dark pink), making synapses with mitral cells and olfactory neurons in the GL. (Right panel) Fluorescent SVZ-derived inhibitory interneurons are visible in the OB 14 days after in vivo electroporation of a GFP-encoding plasmid in the SVZ. DG dentate gyrus, OB olfactory bulb, RMS rostral migratory stream, SVZ subventricular zone, GCL granule cell layer, MCL mitral cell layer, GL glomerular layer
Many studies have conclusively proven the existence of neurogenesis in the human brain (Goritz and Frisen 2012; Spalding et al. 2013). Whereas the RMS is highly prominent in rodents, its presence in the adult human brain is still a subject of controversy (Curtis et al. 2007b; Sanai et al. 2007). A recent report monitoring levels of nuclear bomb test-derived 14C in genomic DNA has shown very limited olfactory bulb neurogenesis in the adult human brain (Bergmann et al. 2012). However, a prominent RMS is visible in the human infant brain up to 18–20 months after birth (Sanai et al. 2011). The extensive migration of neural progenitors observed in this early postnatal stage could be linked to the need to maintain a high level of functional synaptic plasticity at this crucial stage of human development. Indeed, it has been suggested that this migration may also be the target of a number of neurological conditions developing in early infancy (Sanai et al. 2011).
Interestingly, several rodent and human studies have shown that SVZ-derived neuroblasts have the ability to be re-routed to sites affected by injury, trauma, stroke or neurodegeneration (Curtis et al. 2007a; Sundholm-Peters et al. 2005; Arvidsson et al. 2002; Ohab et al. 2006). Studying neuroblast motility may therefore be therapeutically relevant not only for devising endogenous stem cell-based repair strategies but also to promote recruitment of transplanted progenitors in neuroregenerative approaches. Finally, many factors and intracellular regulators of neuroblast migration may also influence pathological cell migration, as in the case of highly metastatic brain tumour cells. This chapter provides an overview of the main extracellular factors regulating RMS neuroblast migration in the postnatal brain.
2 The Migration of SVZ-Derived Neuroblasts
RMS neuroblasts display a very characteristic mode of migration, which does not rely on other cell types (like, for example, glial-guided or axon-guided migration in the developing brain) (Lois et al. 1996). Indeed, neuroblasts slide along each other in chains, which can be visualized by immunostaining for neuroblast markers like the polysialilated form of neural adhesion molecule (PSA-NCAM) (Fig. 9.7a). The integrity of the chains is also favoured by the presence of an astroglial “tunnel” encasing the RMS (Fig. 9.1c), which acts not only as a physical barrier but also as a signaling system contributing to efficient migration (see also below) (Bozoyan et al. 2012). In the intact brain, a complex balance of chemoattractant, chemorepellent and motogenic factors ensures the directed migration of RMS neuroblasts towards the OB, even though the presence of the OB is not absolutely necessary for the directed migration of neuroblasts (Kirschenbaum et al. 1999). The typical chain migration can be recapitulated in vitro by embedding RMS explants in a three-dimensional Matrigel matrix (Wichterle et al. 1997) (Fig. 9.7g), showing that neuroblasts have an intrinsic capacity to migrate even when they are isolated from their native environment.
Time-lapse imaging studies have highlighted distinct phases in neuroblast migration (Nam et al. 2007; Schaar and McConnell 2005) (see also Chaps. 1, 4, 6 and 7) (Fig. 9.2): (1) extension of a leading process in the direction of migration, followed by its stabilization via contacts with other cells and/or the extracellular matrix; (2) formation of a dilation in front of the nucleus, where membranous organelles and the centrosome are located, and where endocytic trafficking weakens adhesion; (3) advancement of the nucleus in the dilation, helped by myosin II-mediated contraction at the cell rear (nucleokinesis step). Continuous repetition of this cycle results in the forward movement of neuroblasts.
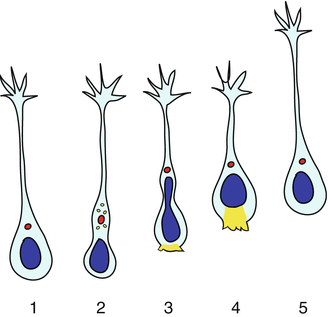
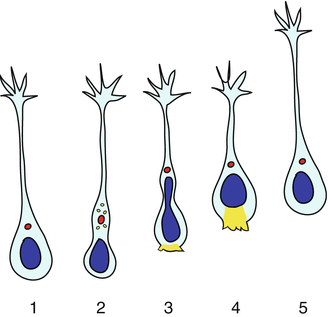
Fig. 9.2
Stereotypical phases of RMS neuroblast migration. 1 Neuroblasts extend a leading process tipped with a dynamic growth cone forming transient adhesions. 2 The process stabilizes by strengthening adhesions and a dilation forms in front of the nucleus containing trafficking vesicles (yellow dots) and the centrosome (red dot) connected to microtubules encasing the nucleus (not shown). 3–4 Endocytic events in the dilation weaken adhesion, while myosin II-mediated contraction (yellow shading) at the cell rear promotes nucleokinesis. 5 The cell starts the cycle again by extending a new leading process
Localization of the centrosome in front of the nucleus and the establishment of a protrusion in the direction of the OB are key events requiring the involvement of polarity regulators, such as the Partitioning defective (Par) proteins (Goldstein and Macara 2007). Among these, Par1/MARK2 is required for polarizing the neuroblast leading process, thus contributing to the directed migration towards the OB (Fig. 9.3). Depletion of MARK2 via shRNA disrupts migration directionality and results in poor integration of interneurons in the OB (Mejia-Gervacio et al. 2012). MARK2 may function by phosphorylating several microtubule-associated proteins such as tau, or MAP2/4 and doublecortin (DCX), a crucial molecule required for both stabilization of the leading process and nuclear translocation. Indeed, DCX suppresses secondary branching and promotes coupling between nucleus and centrosome during migration (Koizumi et al. 2006). The latter role may involve the coordination of DCX function with the dynein protein complex. Given that the centrosome acts as an anchor to the minus end of microtubules encasing the nucleus, dynein could promote translocation of the nucleus via its minus end-directed motor activity. Indeed, disruption of dynein or dynein-associated components such as Lis1 or Ndel1 impairs nucleus-centrosome coupling and ultimately affects neuronal migration (Shu et al. 2004; Tanaka et al. 2004) (see also Chaps. 1 and 7).
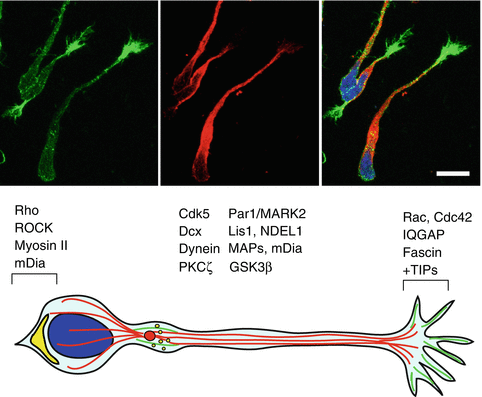
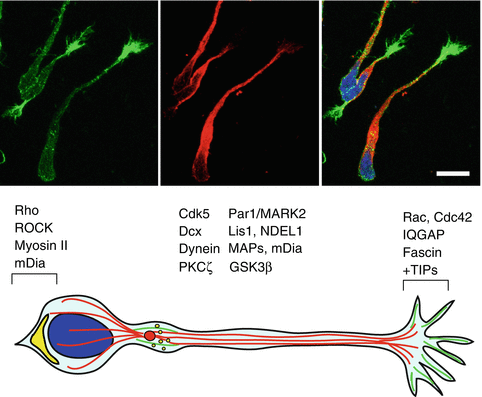
Fig. 9.3
Intracellular mechanisms regulating RMS neuroblast migration. (Top) Confocal images of postnatal rat migratory neuroblasts. Microtubules (visualized by βIII tubulin immunostaining, red) fill in the neuroblast leading processes, which are tipped with a growth cone-like structure enriched in actin filaments (visualized by fluorescent phalloidin, green). Cell nuclei are stained with Hoechst (blue). Scale bar, 10 μm. (Bottom) Schematic diagram showing important intracellular regulators of neuroblast migration. The microtubule cytoskeleton (red lines) is necessary for maintenance and growth of the leading process, whereas an intact actin cytoskeleton (green) is necessary for repolarization and centrosome reorientation, which is dependent on GSK3β and PKCζ activity. Par1/MARK2 phosphorylates MAPs and regulates the activity of other microtubule regulators, such as DCX and Cdk5. DCX may also promote coupling between nucleus and centrosome, together with the Lis1/Ndel1/dynein complex. Cdk5 contributes to the dynamic organization of the microtubule cytoskeleton by phosphorylating a number of substrates including Ndel1, DCX, and FAK. Centrosomally anchored microtubules may become captured in the leading process through interactions between the Rac1/Cdc42 effector IQGAP and plus-end MT tip proteins. Actomyosin-based contractility mediated by mDia and ROCK at the cell rear (yellow) promotes nucleokinesis. The actin-bundling protein fascin regulates actin bundling in peripheral filopodia, and may participate in controlling adhesion during migration. Localized clathrin-mediated endocytosis of adhesion components (yellow dots) occurs in the dilation forming ahead of the nucleus after extension of the leading process, weakening adhesion before nucleokinesis
Par1/MARK2 may also regulate Cyclin-dependent kinase 5 (Cdk5), another crucial molecule regulating the tangential migration of neuroblasts along the RMS. Cdk5 deletion impairs chain formation, speed, directionality and leading process extension of SVZ-derived neuroblasts in a cell-autonomous manner (Hirota et al. 2007). This important kinase may contribute to the dynamic organization of the microtubule cytoskeleton by phosphorylating a number of substrates involved in neuronal migration, such as Ndel1, Pak1, CRMP-2, DCX, and FAK (Hirota et al. 2007).
Also involved in the directed migration of neuroblasts is protein kinase C ζ (PKCζ), a member of the Par3-Par6-PKCζ complex, a master regulator of polarization in a variety of cellular contexts (McCaffrey and Macara 2009). Upon binding of active Cdc42 to Par6, activation of the polarity complex results in PKCζ-mediated phosphorylation and inhibition of glycogen synthase kinase 3β (GSK3β), ultimately affecting proper centrosome localization and microtubule stability. Indeed, both GSK3β and PKCζ inhibition block Slit-induced reorientation of the centrosome and process stabilization (Higginbotham et al. 2006), suggesting a conserved role for these molecules in the regulation of neuroblast polarization. Importantly, disruption of the actin but not microtubule cytoskeleton impaired centrosome movement within the cell body. This suggests that while an intact microtubule cytoskeleton is necessary for maintenance and growth of leading process, an intact actin cytoskeleton is necessary for repolarization and centrosome reorientation.
The centrosomally anchored microtubules may become captured at the edge of the leading process through interactions between the Rac1/Cdc42 effector IQGAP and plus-end MT tip proteins. Consistent with this model, IQGAP is required for neuroblast motility (Balenci et al. 2007). The essential role of tightly regulated actin dynamics for migration along the RMS is also emerging from studies in knockout mice lacking actin regulators, such as the Rho effectors mDia1 and mDia3 and the actin-bundling protein fascin. Both animal models have an abnormal RMS with a caudal accumulation of migratory neuroblasts and a smaller OB (Shinohara et al. 2012; Sonego et al. 2013). mDia is required for anterograde F-actin movement towards the leading process during nucleus-centrosome separation and for the formation of an “F-actin cup” at the cell rear during nuclear translocation, probably by generating actin filaments in actomyosin bundles for nuclear translocation. At the cell rear, activation of Rho-associated protein kinase ROCK downstream of Rho would lead to phosphorylation and activation of myosin light chain kinase (MLCK), thus promoting actomyosin-based contractility and nuclear movement over large distances (Shinohara et al. 2012), as shown in cortical interneurons (Godin et al. 2012). Instead, F-actin condensation ahead of the nucleus may ensure centrosomal movement before nuclear translocation. The same area displays an accumulation of fascin, an actin bundling protein also localized on peripheral filopodia of the leading process tip. Consistent with a role in regulating the directed motility of RMS neuroblasts, fascin depletion disrupts neuroblast morphology, causing ectopic branching and impairing migration both in vitro and ex vivo (Sonego et al. 2013). The ability of fascin to cycle on-off actin filaments via PKC-dependent phosphorylation on Ser39 is crucial for neuroblast migration, and may provide a molecular link between actin and adhesion dynamics (Anilkumar et al. 2003).
Together with cytoskeletal regulation, membrane trafficking is likely to play an important role in the directed migration of neuroblasts. Clathrin-mediated endocytic events frequently occur in the dilation forming ahead of the nucleus after extension of the leading process (Shieh et al. 2011). Localized endocytosis of adhesion components such as β1 integrins (see also below) or N-cadherin (Kawauchi et al. 2010) could weaken adhesions to facilitate forward translocation during neuronal migration. Blocking endocytosis by pharmacological inhibition or expression of either dominant negative dynamin or clathrin impairs migration by affecting the ability of the cell body to advance. From these experiments a model emerges where anchoring adhesions in the leading growth cone provide traction forces, a gradient of adhesive strength enables the cell rear to detach from the substrate during somal translocation, while endocytosis in the dilation weakens adhesive contacts and prepares the cell for the nuclear translocation into the dilation (Shieh et al. 2011). Recent studies on embryonic neuronal migration have started to highlight the importance of Rab GTPases in trafficking of cell adhesion molecules (Kawauchi et al. 2010), which could play similar roles also in postnatal neuroblast migration. Exploring the function of these and other membrane traffic regulators will help us understand how localized signalling is achieved during the polarized migration of postnatal neuroblasts and how this can be coordinated with cytoskeletal and centrosome dynamics.
3 Factors Controlling RMS Neuroblast Migration
The directed migration of RMS neuroblasts relies on the complex combination of a variety of extracellular factors with chemorepellent, chemoattractant, and motogenic activities. In the last two decades in vitro migration assays using RMS explants and a variety of transgenic mouse models have been instrumental to identify several regulators of neuroblast migration, including cell adhesion/ECM and axon guidance molecules, growth factors, and neurotransmitters (Figs. 9.4, 9.5, 9.6 and 9.8). The following section describes the roles of key extracellular signals controlling the different phases of neuroblast migration, including detachment from the SVZ, tangential migration along the RMS, and the final switch to radial migration in the OB.
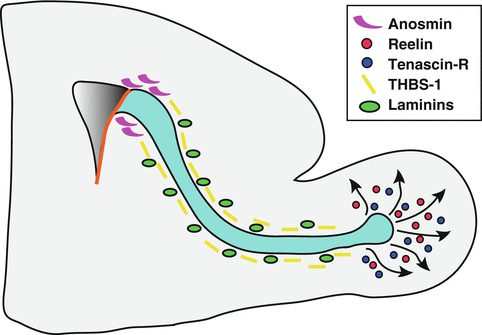
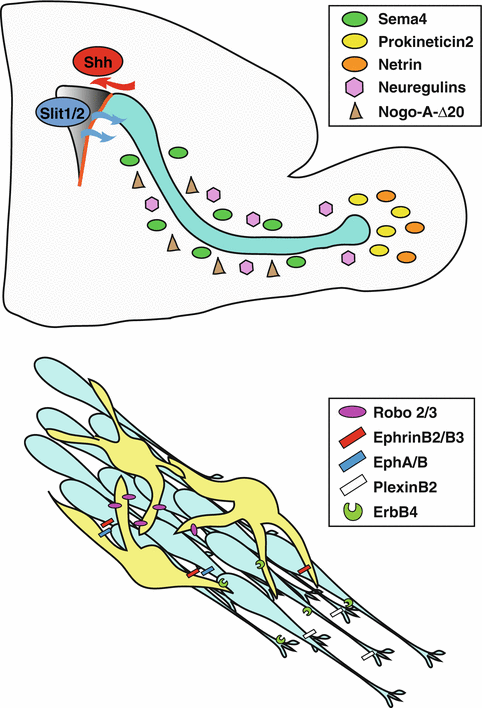
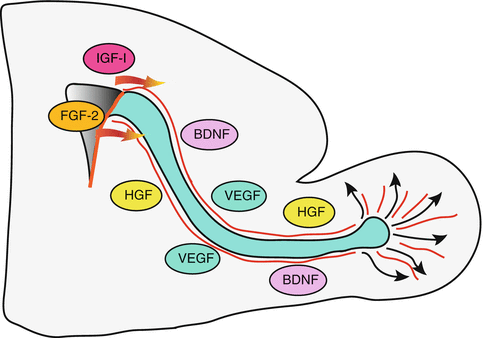
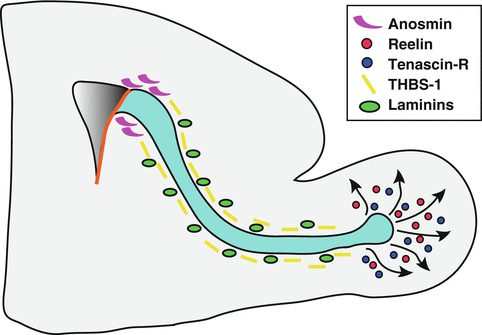
Fig. 9.4
Cell adhesion and ECM molecules involved in neuroblast migration. PSA-NCAM and various integrin subunits modulate neuroblast motility along the RMS. Integrins can interact with a number of laminins present along the RMS. The ECM glycoprotein anosmin secreted by neuroblasts may cooperate with FGFR1 signalling to promote detachment of neuroblasts from the SVZ. Secreted thrombospondin-1 (THBS-1) and Reelin act on the same receptors (ApoER2 and VLDLR) to modulate tangential and radial migration, respectively. Expression of the ECM component Tenascin-R is activity-dependent and controls chain dispersion in the OB
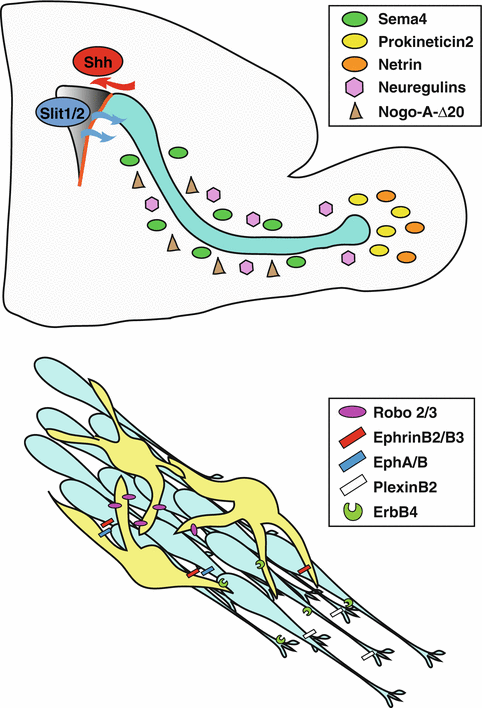
Fig. 9.5
Axon guidance molecules regulating neuroblast migration. (Top) Slits1/2 derived from the choroid plexus function as chemorepellents for RMS neuroblasts, while Sema4 acts on PlexinB2 receptors to restrict tangentially migrating neuroblasts to the RMS, forcing cells to move along the caudo-rostral axis. The Nogo-A-Δ20 domain may contribute to ensure proper adhesion/detachment cycles in neuroblasts along the RMS. Prokineticin 2 expressed in the OB acts as a chemoattractant for neuroblasts along the RMS and as a dissociation signal at the OB entry. Netrin secreted by mitral cells in the OB may promote the directed migration of neuroblasts expressing the DCC receptor. (Bottom) Axon guidance molecules also function in neuroblast-astrocyte cross-talk along the RMS. Neuroblasts secrete Slit-1 to remodel the surrounding glial tubes via Robo2/3 receptors present on astrocytes. Eph receptors in the RMS may act upon binding of EphrinB2/B3 ligands present on astrocytes to regulate chain maintenance. NRG/ErbB4 signalling regulates neuroblast-astrocyte interaction, chain organization and both tangential and radial migration
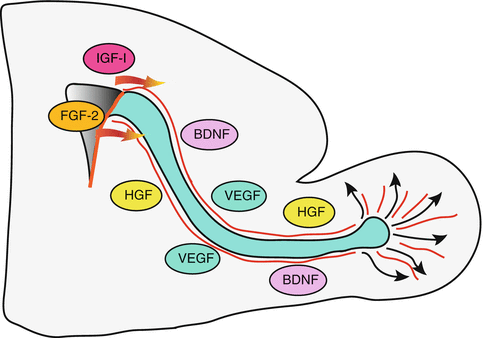
Fig. 9.6
Growth factors involved in neuroblast migration. IGF-I and FGF-2 promote the exit of neuroblasts from the SVZ while VEGF may favour tangential migration via VEGFR2. VEGF secreted by astrocytes may also indirectly regulate the motility of neuroblasts by remodeling blood vessels functioning as scaffold for migration. BDNF secreted by blood vessels promotes neuroblast motility via p75NTR, while HGF acts as a motogenic factor by binding the Met receptor present on neuroblasts. See text for details
3.1 Adhesion Molecules/ECM Molecules
PSA-NCAM: A function for PSA-NCAM in the formation of neuroblast chains was initially suggested based on the high expression of this molecule by migrating neuroblasts (Rousselot et al. 1995). Indeed, migration along the RMS is hampered in mice lacking NCAM and PSA (Tomasiewicz et al. 1993; Cremer et al. 1994). Although chains are still visible in NCAM −/− mice, they appear disorganized and more sparse compared to wild-type animals (Chazal et al. 2000). However, migration may rely on PSA-NCAM more in the early postnatal RMS compared to later stages. PSA-NCAM may actually serve more as an important mediator in favouring the interaction of neural progenitors with their environment, rather than between migrating precursors themselves. Indeed, enzymatic removal of PSA by intraventricular injection of neuraminidase causes a major disorganization of the RMS, disruption of astrocyte/neuroblast alignment and most of all enhances dispersion of cells into neighbouring areas, like the striatum or the cortex (Battista and Rutishauser 2010). This is likely due not only to abnormalities in the astrocytic “tunnels” encasing the neuroblast chains, but also to the unveiling of some new, PSA-independent migratory properties coupled with NCAM-dependent triggering of neuroblast differentiation.
Integrins: Several studies have confirmed the presence of different integrins along the RMS, even though a thorough characterization of the range of integrins present at different ages is still lacking. For example, in neonatal mice α1 integrin subunit is expressed along the entire stream, but then is downregulated from early postnatal stages onwards (Murase and Horwitz 2002). In contrast, the β8 integrin subunit is expressed throughout development and adulthood and its genetic deletion causes severe defects in chain migration, with the appearance of disorganized neuroblast clusters and increased numbers of GFAP-positive astrocytes throughout the brain (Mobley and McCarty 2011). αv, β3 and β6 subunits are observed along the RMS from early postnatal/young adulthood stages onwards (Murase and Horwitz 2002). The presence of different integrin subunits in the RMS may reflect the diverse range of extracellular matrix (ECM) components surrounding the neuroblast chains. Indeed, integrins act as receptors for several laminins, which also display a specific distribution in the different cell types composing the SVZ niche (Kazanis et al. 2010). In the RMS, prominent expression of several α, β and γ laminin subunits can be detected, including α1, α2, and α4 (Belvindrah et al. 2007). While the requirement of many laminin subunits for neuroblast migration is difficult to test due to the early lethality of knockout mouse models (such as α1, β1 and γ1-deficient mice), α2/α4 double knockout animals could be examined thanks to their ability to survive to adulthood. These mice display a less compact RMS, consistent with the idea that laminin/integrin signaling ensures the formation of organized neuroblast chains. Since the α2 and α4 subunits are components of several laminin isoforms (laminin-2, -4, -12 for α2 and laminin-8 and -9 for α4, respectively) and given the lack of RMS defects in the single α2 and α4 knockouts, it is likely that several laminin isoforms perform redundant roles in the maintenance of neuroblast chains (Belvindrah et al. 2007).
Genetic deletion or blocking of β1 integrin disrupts RMS architecture, causing defects in the glial tubes surrounding neuroblast chains. As a consequence, ectopic migration is observed into the tissue surrounding the RMS and also the medial side of the lateral ventricle, an area usually almost completely lacking migratory neuroblasts (Kazanis et al. 2010). However, quite surprisingly cells lacking β1 are still able to extend dynamic protrusions and migrate towards the OB. The disruption in chain migration caused by blocking or genetically ablating β1 suggests that this subunit is specifically required to ensure proper cell-cell interactions during migration (Emsley and Hagg 2003; Belvindrah et al. 2007). In absence of β1 integrin, cells no longer form compact chains also in vitro, and this influences their mode of migration, which becomes more random and less directional. The crucial role of the β1 subunit suggests that several laminin-binding integrins expressed on neuroblasts (such as α1β1, α2β1, α3β1, α6β1, and α7β1) may cooperate with each other, with additional ECM and other secreted signaling molecules/surface receptors to ensure migration of neuroblasts in compact chains (Belvindrah et al. 2007). Interestingly, integrins can also control the activity of N-cadherin, a cell adhesion molecule highly expressed along the RMS and downregulated in the OB, where cells radially disperse (Yagita et al. 2009). In this regard, it is also worth pointing out that gangliosides such as ganglioside 9-O-acetyl GD3 (9acGD3) are markedly expressed in the RMS from embryonic until adult stages. This acetylated ganglioside displays a punctate distribution along the surface of cultured neuroblasts, which partially colocalizes with β1 integrin (Miyakoshi et al. 2012). Even though blocking 9acGD3 in vitro does not disrupt neuroblast chains, it decreases the speed of migration and leads to chain retraction, suggesting that 9acGD3 could regulate neuroblast motility by modulating integrin-dependent adhesion. Interestingly, the deacetylated form of GD3 can interact with Tenascin-R (Probstmeier et al. 1999), another ECM molecule regulating the radial migration of neuroblasts in the OB (see below), which highlights the potential ability of this glycolipid to mediate distinct modes of migration when interacting with different adhesion molecules present in the extracellular environment.
Galectin-3: Galectin-3 is a member of a family of 15 proteins identified by their similar carbohydrate recognition domains, with a conserved sequence of approximately 130 aminoacids able to bind galactoside residues. Among galectins, Galectin-3 can regulate cell-ECM interactions through laminin and integrins, but is also able to shuttle between the cytoplasm and the nucleus, where it regulates pre-mRNA splicing (Elola et al. 2007). Interestingly, Galectin-3 is expressed selectively in the RMS but not in the OB, suggesting a specific role in tangential migration. More detailed analysis showed that it can be found in GFAP+ astrocytes and ependymal cells of the SVZ and in the astrocytes lining the RMS, but is excluded from PSA-NCAM+ migratory neuroblasts. Gal3 ko mice display distorted and thicker process of GFAP+ cells, but chain integrity is not altered, even though the number of individually migrating cells appears increased. However, BrdU labeling experiments clearly showed a significantly impaired neuroblast migration, resulting in a marked decrease of neuron number in the OB (Comte et al. 2011). Galectin-3 may regulate neuroblast migration in several ways, given its ability to bind β-galactoside residues on Epidermal Growth Factor Receptor (EGFR), laminin, integrins, NCAM and tenascin, all involved in controlling neuroblast motility. First, the lack of Galectin-3 decreases and disrupts cilia, crucial structures present in the ependymal cells lining the wall of the ventricle. The correct orientation and beating of ependymal cilia ensures proper flow of cerebrospinal fluid (CSF), establishing gradients of chemorepellents in the SVZ regulating the directed migration of neuroblasts (Sawamoto et al. 2006), which may therefore be affected by the deletion of Galectin-3. Moreover, the disrupted astrocytic tunnels observed in Gal3 ko mice are likely to have a profound effect on neuroblast migration, given that these structures play a fundamental role not only in physically constraining the migratory neuroblasts, but also in controlling the speed of migration through important cell-cell interactions (see also below). Finally, Galectin-3 may also decrease the activation state of epidermal growth factor receptor (EGFR), which inhibits neuronal migration (Kim et al. 2009). It will be important to investigate the potentially multiple signaling pathways operating downstream of Galectin-3 in the control of neuroblast motility.
Thrombospondin-1 (THBS-1): Thrombo-spondins are secreted proteins involved in cell-cell and cell-matrix interactions in a variety of cellular contexts. Thrombospondin-1 (THBS-1) can interact with integrins, proteoglycans, and growth factors like PDGF and TGFβ (Lawler 2000), and is found in both the SVZ and the RMS. THBS-1 contributes to the stabilization of RMS chains by binding to apolipoprotein E receptor 2 (ApoER2) and very low-density lipoprotein receptor (VLDLR) present on migrating neuroblasts. Addition of THBS-1 to RMS explants increases chain length, while THBS-1 ko mice exhibit higher number of individual migrating cells in vitro, a widened, less compact RMS, and a delayed migration to the OB in vivo (Blake et al. 2008). However, the RMS phenotype in THBS-1 ko is milder than that observed in mice lacking both ApoER2 and VLDLR, where chain formation is completely absent (Andrade et al. 2007), suggesting that other extracellular factors may act on ApoER2 and VLDLR in the RMS. Remarkably, while ApoER2 and VLDLR maintain chains upon binding THBS-1 along the RMS, in the OB they promote chain dispersion and radial neuroblast migration downstream of Reelin (Herz and Chen 2006; Hack et al. 2002). Intriguingly, the interaction of ApoER2 and VLDLR with both THBS-1 and Reelin lead to a common initial event, the intracellular phosphorylation of the adaptor protein Disabled (Dab1). How the ApoER2 and VLDLR downstream signaling pathways diverge in mediating such different effects (tangential versus radial migration) is still unclear.
Reelin: Reelin is a large secreted glycoprotein essential for the development of laminated structures in the brain (Tissir and Goffinet 2003), and is best known for its role in promoting radial neuronal migration in the neocortex and in the hippocampus. In the postnatal brain, Reelin causes the detachment of RMS neuroblast chains, allowing proper radial migration within the OB (Hack et al. 2002). Consistent with this function, Reelin mutant animals (Reeler mice) display abnormal neuroblast accumulation at the end of the RMS, pointing to a severe radial migration defect resulting in the loss of the stereotypical layer organization of the OB and in an overall significant reduction of newly generated neurons in this structure (Hack et al. 2002; Kim et al. 2002). Reelin is highly expressed by mitral cells, the principal neuronal targets for the interneuron precursors that arrive via the RMS in the OB. Moreover, a descending Reelin protein gradient can be detected from the mitral cell layer (MCL) through the entire granule cell layer (GCL) as far as the RMS, which instead is negative for reelin. A recent study has proposed that reelin, which has a higher affinity for ApoER2 and VLDLR than THBS-1, might displace THBS-1 and other putative ligands from ApoER2 and VLDLR once neuroblasts start to enter the OB, resulting in the dissolution of chains and helping achieve correct positioning of cells in the OB layers (Blake et al. 2008). In vitro studies using neuroblast cultures suggest that reelin may induce neuroblast detachment via a Dab1/PI3K pathway leading to MAPK activation and ERK phosphorylation (Simo et al. 2007), but the functional relevance of these events still needs to be explored in vivo.
Tenascin-R: Among the members of the tenascin gene family is Tenascin-R, an ECM component containing a cysteine-rich amino terminal region, epidermal growth factor (EGF)-like domains, fibronectin type III homologous repeats and a domain homologous to fibrinogen (Jones and Jones 2000). This molecule promotes the detachment of neuroblasts from chains at the end of the RMS and their radial migration in the OB. Consistent with this function, Tenascin-R is detectable exclusively in the deep layers of the OB and around the rostral section of the RMS. TNR ko mice have normal RMS architecture and tangential migration, but display neuroblast accumulation at the rostral RMS when cells enter the OB, indicating a failure in chain dispersion. Interestingly, expression of Tenascin-R is activity-dependent, as it is significantly reduced by odor deprivation (Saghatelyan et al. 2004). Tenascin-R therefore may act as an important signal linking network activity with recruitment of newborn neurons in the OB. The Tenascin-R receptors involved in this function remain to be identified. They could include contactin (or F3) (Pesheva et al. 1993), acetylated gangliosides like GD3 (Probstmeier et al. 1999), and receptor protein-tyrosine phosphatases belonging to the family of chondroitin sulfate proteoglycans (CSPGs) (Xiao et al. 1997). Another possible mode of function may involve the ability of Tenascin-R to capture and present extracellular factors promoting radial migration. In this regard, the possibility of cross-talk between Tenascin-R and the reelin signaling pathway, also involved in radial migration in the OB, remains to be investigated. Interestingly, grafting experiments of Tenascin-R-expressing cells showed that this molecule not only promotes the detachment of neuroblasts from chains, but is also sufficient to re-route tangentially migrating neuroblasts towards non-neurogenic areas (Saghatelyan et al. 2004), which could become an important aspect in therapies exploiting endogenous stem cell repair.
3.2 Axon Guidance Molecules
Slit/Robo: Slits are diffusible proteins involved in multiple aspects of neurodevelopment, such as axon guidance and neuronal migration (Ypsilanti et al. 2010). Two Slit members, Slit1 and Slit2 are expressed in the adult brain septum and choroid plexus and are sufficient to repel SVZ-derived neuroblasts in vitro (Hu 1999; Wu et al. 1999). Further studies using Slit1 and Slit2 ko mice identified the nature of the septum-derived chemorepulsive activity as a mixture of Slit1 and Slit2, while Slit2 is the choroid plexus-derived chemorepulsive factor (Nguyen-Ba-Charvet et al. 2004), demonstrating that both Slits play an important role in orienting the migration of SVZ-derived neuroblasts. However, Slits do not seem to act purely as chemorepellents. Indeed, Slit1 is also present in type C and A cells in the SVZ/RMS, while is absent from GFAP+ astrocytes. In Slit1 ko mice, neuroblasts leave the RMS prematurely to migrate dorsally and caudally to the SVZ, throughout the corpus callosum (Nguyen-Ba-Charvet et al. 2004). In the same study, genetic deletion of Slit1 markedly increased the migration of neuroblasts out of SVZ explants, leading the authors to propose a cell-autonomous role for Slit in neuroblast migration. A second report more directly analyzed the dynamics of Slit1 ko neuroblasts labeled with DiI in brain slices and found that they were 40 % slower than wild-type, consistent with a marked reduction in the proportion of cells able to reach the OB (Kaneko et al. 2010). Slit1 ko neurons transplanted in a wild-type RMS showed significantly slower speed compared to Slit1-expressing wild-type cells, supporting a cell-autonomous role for Slit1 in neuroblast migration. In addition, Slit1-expressing wild-type cells displayed a slower and more irregular migration when transplanted in a Slit1-deficient RMS compared to a wild-type RMS, revealing also a non-autonomous function for Slit-1 in neuroblast migration. Interestingly, the Slit receptors Robo2 and Robo3 are present on both migrating neuroblasts and on the surrounding astrocytes in the RMS. Elegant experiments have shown that Slit1 secreted by the migrating neuroblasts “repels” and changes the morphology of the surrounding astrocytes via Robo signaling, thus contributing to the formation and maintenance of the astrocytic tunnels that ensure proper long-range migration (Kaneko et al. 2010).
Slit can also bind the secreted factor Netrin-1, which is present on migrating neuroblasts together with its receptor DCC (Murase and Horwitz 2002) (see below). In other contexts, Slit can modulate DCC-mediated responses by silencing Netrin-1 signalling, but it is still unclear whether such mode of action is preserved along the RMS. It is intriguing that while all PSA-NCAM+ cells in the RMS express Slit1, radially migrating cells in the OB appear to downregulate Slit1 levels, suggesting that the switch from tangential to radial migration may involve modulation of Slit signaling. The absence of Slit favours independent migration of neuroblasts, and a possible cross talk with other signaling molecules promoting the dispersion of chains in the OB such as reelin or Tenascin-R would be worth investigating.
Netrin-1/Dcc: Both Netrin and the netrin receptors neogenin and DCC are strongly expressed by migrating neuroblasts in the RMS during E15 until P5, a time of massive migration from the SVZ to the OB (Murase and Horwitz 2002). Moreover, mitral cells express high levels of netrin-1, at least in late embryonic and early postnatal stages, but downregulate netrin-1 after P5. This suggests that a chemogradient of netrin-1 secreted by the mitral cells may help guiding neuroblast migration at least in the early postnatal brain. Time-lapse imaging experiments in brain slices performed in the presence of a function-blocking antibody against the netrin receptor DCC show a loss of neuroblast directionality and a significant decrease in their speed, pointing to a role for the Netrin-DCC signaling system in regulating the directed migration of neuroblasts along the RMS. A more recent report has highlighted the presence of high levels of netrin-4 in some astrocytes located in the OB and along the border of the anterior RMS in adult mice (Staquicini et al. 2009). These netrin-4-producing astrocytes may have a role in restricting the migratory pathway, promoting the entry of neuroblasts into the OB. However, whether this is indeed the case or whether there may be an additional role for netrin-4 in controlling the proliferation of migratory neuroblasts remains to be investigated.
ErbB4/Neuregulin NRG1-NRG3: Neuregulins (NRGs) are multiple EGF-like domain-containing ligands, which act on receptor tyrosine kinases ErbB2, ErbB3 and ErbB4. NRGs are well-characterized regulators of synaptic plasticity (Buonanno 2010), oligodendrocyte/Schwann cell lineages (Garratt et al. 2000), and muscle spindle formation (Hippenmeyer et al. 2002). Moreover, they can also control glial-guided neuronal migration in the developing cortex and cerebellum (Anton et al. 1997; Rio et al. 1997). NRG1-3 are all expressed in the postnatal CNS and they activate ErbB4, which is selectively expressed at high levels in the SVZ and RMS, mainly in type A migratory neuroblasts, a subset of GFAP+ astrocytes and type C proliferating progenitors (Anton et al. 2004). Conditional deletion of ErbB4 in nestin-expressing stem cells or in GFAP-expressing stem cells and astrocytes leads to similar phenotypes displaying fragmented neuroblast chains and an RMS with jagged boundaries. The close interaction between neuroblasts and ensheathing astrocytes along the RMS was disrupted when ErbB4 was deleted in GFAP-expressing cells, suggesting that this receptor is required in both neuroblasts and astrocytes to ensure proper formation and organization of the RMS in vivo. Live cell-imaging also revealed impaired orientation and decreased speed of neuroblast migration along the RMS in Erbb4 lox/-;hGFAP-Cre mice. The impaired migration in Erbb4 lox/-;hGFAP-Cre mice leads to disrupted organization of the OB, which displays a reduction in interneuron number, abnormal interneuron morphology and distribution and a tendency of cells to accumulate at the end of the RMS in the internal granular layer (Anton et al. 2004). Together, these observations indicate that lack of ErbB4 affects both tangential and radial migration. Immunolocalization analysis of the different NRGs suggests that NRG1 type III isoform (containing cysteine-rich/SMDF domains) is the predominant ErbB4 ligand acting in the RMS, where it is detectable especially in early postnatal stages. Indeed, NRG1 exhibits almost complete overlap with PSA-CAM+ neuroblasts in the RMS (Ghashghaei et al. 2006). Consistent with this, in vitro stripe assays indicate that NRG1 type III on the cell surface may provide a permissive guidance substratum with a motogenic effect for ErbB4+ migrating neuroblasts (Anton et al. 2004). Other NRGs such as NRG2 and NRG3 are mostly expressed in the OB, where they could influence the radial migration and final differentiation of neuroblasts. How ErbB4 signalling system can influence migration is still unclear, but possible mechanisms include its ability to cross-talk with integrin-linked pathways and to regulate transcription following proteolysis and entry into the nucleus of its cytoplasmic tail.
Ephrin-B2/EphB2: The Eph family of receptor tyrosine kinases and their transmembrane-associated ephrins are divided into two subclasses, A and B, based on their binding specificities. During development Eph/ephrin signalling contributes to the regulation of a multiple events such as axon guidance and neural crest cell migration (Klein 2012). Eph receptors signal through their tyrosine kinase domain upon ligand binding (Hall and Lalli 2010). However, Ephrin-B ligands also transduce intracellular signals through their cytoplasmic tyrosine residues, thus allowing bidirectional signaling (Holland et al. 1996). EphB/EphA4 and ephrin-B bi-directional signaling favours cell-cell repulsion, for example to restrict cell intermingling during boundary formation. However, it may also mediate attraction in other contexts such as endothelial assembly in blood vessels (Yancopoulos et al. 1998). Ephrin B2 and B3 ligands are present on astrocytes in both the SVZ/RMS and OB. Infusion of truncated EphB2 and ephrin-B2 proteins into the lateral ventricle to perturb normal EphB/ephrin-B signaling increases cell proliferation in the SVZ and disrupts neuroblast chain migration (Conover et al. 2000). Since Ephrin ligands are present on astrocytes both in the SVZ and along the RMS, they may help to restrict the migratory path that also contains the ephrin receptors EphA4, B1 and B2. It is still unclear which of these receptors are actually expressed by the migratory neuroblasts in the RMS, and whether disruption of chain migration may be a secondary effect following the abnormal proliferation caused by disruption of ephrin signaling in the SVZ. However, the presence of both ephrin ligands and Eph receptors along the RMS migratory route suggests that this system plays an active role in regulating cell-cell signaling both during proliferation in the SVZ and in migration towards the OB. The molecular details of this event and the functional role of a potential Ephrin-B/EphB bidirectional signaling in this process remains to be clarified.
Semaphorin/Plexin-B2: Semaphorins are axon guidance molecules controlling neuronal migration in the developing nervous system (Renaud et al. 2008; Kerjan et al. 2005; Tran et al. 2007). They act by binding to Plexin receptors, which are divided into four subgroups (A-D) based on structural features. Type B Plexins are expressed in the postnatal CNS but their function is still largely unclear. A recent report analyzing Plxnb2 ko mice highlighted an important role for this semaphorin receptor in postnatal neurogenesis. In the SVZ, Plexin-B2 is highly expressed in GFAP+ astrocytes and Mash1+ transit amplifying progenitors. In the RMS, Plexin-B2 is present in migrating neuroblasts but absent in the surrounding astrocytic tunnels. Interestingly, in the OB Plexin-B2 expression is not detectable in the radially migrating neuroblasts cells, while it is found at lower levels in all periglomerular cells, mitral cells and olfactory axons (Saha et al. 2012). The presence of the class IV Semaphorins (Sema4A, 4C, 4D, 4G) acting as ligands for Plexin-B2 along the RMS, granular cell and mitral cell layers suggests that a classical Semaphorin-Plexin signaling may provide a way to fine-tune neuroblast migration in “decision points” such as the RMS exit and the different layers of the OB. Indeed, besides disruption in proliferation, Plxnb2 ko mice display ectopic neuroblast migration in non-neurogenic areas like the corpus callosum and septum. Moreover, Plxnb2 ko neuroblasts move faster, are less directed and leave the RMS more rapidly once in the OB. Sema-Plexin-B2 interactions may therefore help neuroblasts to inhibit radial migration in the CNS as well as polarize the tangential migration along the RMS according to the so-called “surround repulsion” model (Keynes et al. 1997), forcing cells to move along the caudorostral axis. Plexin-B2 can also bind to receptor tyrosine kinases such as MET and RET and modulate their activation by their respective ligands, Hepatocyte Growth Factor (HGF) and Glial cell Derived Neurotrophic Factor (GDNF), two growth factors also involved in neuroblast migration (see below). However, Plexin-B2 does not seem to be necessary for HGF or GDNF-induced neuroblast migration, at least in vitro (Saha et al. 2012).
3.3 Other Factors
Sonic Hedgehog (Shh): The morphogen Shh has recently been implicated in the regulation of neuroblast migration. In vivo perturbation of Shh signaling significantly alters the amount of BrdU+ nuclei found in the SVZ and OB, without altering the total amount of BrdU+ cells. Specifically, overexpression of Shh led to an increase in BrdU+ cells in the SVZ at the expense of the OB, while overexpression of the Shh inhibitor Hip led to opposite effects, suggesting an involvement of Shh signaling in neuroblast proliferation and migration (Angot et al. 2008). Indeed, RMS migratory neuroblasts express both the 12-pass transmembrane protein Patched (Ptc) and the G protein-coupled receptor Smoothened (Smo), which are the main mediators of Shh signaling. In vitro migration assays using RMS explants point to a chemoattractive role for Shh, confirmed by the fact that in vivo grafting of Shh-expressing cells attracts migrating neuroblasts away from the RMS (Angot et al. 2008). Since Shh is highly expressed in the SVZ and in the CSF, this morphogen may act as a molecular cue to control the exit of neuroblasts out of the SVZ, retaining them within the niche. It remains to be seen how Shh exerts this effect, whether classically through attenuation of Gli transcriptional repressors or through modulation of integrin/N-cadherin adhesion, as shown in neural crest cells and in the neuroepithelium (Jarov et al. 2003). Thus, Shh signaling is likely to contribute to the complex balance between attractive, repulsive and motogenic factors regulating the detachment of neuroblasts from the SVZ and the initiation of their migration in the RMS.
Nogo: A recent study has highlighted the contribution of Nogo signaling to the regulation of neuroblast migration. Nogo-A and its receptor NgR1 are well-known regulators of neurite growth and synaptic plasticity in the CNS (Pernet and Schwab 2012), but they have also been implicated in controlling neural stem cell proliferation (Li et al. 2011). Interestingly, while Nogo-A/NgR1 signalling reduces neural stem cell proliferation in vivo, the Nogo-A-Δ20 domain supports tangential migration in the RMS independent of NgR1, which is absent from neuroblasts. This effect appears to rely on the ability to affect cytoskeletal dynamics by stimulating a Rho-ROCK signaling cascade, which is likely to ultimately contribute to the balance of adhesion/detachment cycles during migration (Rolando et al. 2012). Consistent with this, in vivo infusion of a Nogo-A-Δ20 domain blocking antibody causes a marked accumulation of DCX+ neuroblasts in the SVZ, supporting a role for Nogo-A in promoting neuroblast mobilization via its Δ20 domain, facilitating their sliding onto each other. Interestingly, this Nogo-A effect is lost at the rostral end of the RMS, when cells switch from tangential migration to radial migration along vascular scaffolds, suggesting that Nogo-A-Δ20 may act by modulating homotypic interactions between neuroblasts along the RMS. The receptor(s) mediating this Nogo-A-Δ20 dependent effect are still unknown.
Prokineticin 2: Prokineticin (1 and 2) are secreted bioactive molecules acting via two closely related G-protein-coupled receptors (Prokr1 and Prokr2), and are involved in controlling gut motility, reproductive function and circadian output from the suprachiasmatic nuclei (Prosser et al. 2007). Both prokineticin receptor transcripts are highly expressed in the SVZ and RMS, and prokineticin 2 plays an important chemoattractant role for RMS neuroblasts (Ng et al. 2005). Prokr2 is highly expressed by RMS neuroblasts, but is also present on transit amplifying cells, suggesting it could play an additional role in regulating the transition from the transit amplifying “C” cell state to the migratory neuroblast “A” cell state (Puverel et al. 2009). Prokr2 Brdm1 mice (with a null prokineticin receptor 2) show severe defects in neuroblast migration, with substantial accumulation of cells in the RMS and a drastic decrease in the size of the OB, which also displays disrupted organization and failure in differentiation (Prosser et al. 2007). Both Prok2 and Prokr2 genes play an essential role also during the terminal steps of the migration of neuroblast chains in the RMS, since both Prokr2 −/− and Prok2 −/− mice display defective dissociation of chains at the exit of the RMS. These data, together with the specific expression of Prok2 in the OB (Ng et al. 2005) strongly support a role for prokineticin2 as a chemoattractant for SVZ-derived neuroblasts along the RMS and as a dissociation signal at the entry of the OB. Importantly, Prokr1 ko mice retain a normal OB, supporting a specific role for Prokr2 in neuroblast migration. Interestingly, the effects on the OB observed in Prokr2 null mice are more severe compared to those caused by the lack of its ligand Prokineticin2, suggesting that the loss of Prok2 is at least partially compensated in vivo by other mechanisms.
3.4 Neurotransmitters
SVZ and RMS neuroblasts are characterized by a typical “ionic electrophysiological signature” distinct from that observed in mature neurons. Indeed, they have high-input resistances, with an estimated mean resting potential of −59 mV. They also express Ca2+– and voltage-dependent K+ channels and delayed rectifying K+ channels, but they lack inward K+ currents and transient A-type outward K+ currents. About 80 % of RMS neuroblasts express Na+ channels at a lower density than K+ channels (Wang et al. 2003). Expression of Na+ channels by a higher percentage of neuroblasts in the RMS compared to the SVZ suggests that the presence of Na+ channels is an early event characterizing the differentiation of neural progenitors to a migratory phenotype. However, although Na+ channels participate to the generation of action potentials in mature neurons, their function in migrating neuroblasts is still unclear, since these cells are unable to generate action potentials.
Because of the high input resistance observed in neuroblasts, induction of small current flows due to local changes in extracellular signals can significantly modulate cell membrane potentials, which could contribute to influence cell behavior. These current flows may be associated with K+ channel opening/closing or the activation of GABAA receptors, which are found in neuroblasts (Wang et al. 2003; Bolteus and Bordey 2004; Stewart et al. 2002).
GABA is the main inhibitory neurotransmitter in the brain acting through the activation of ionotropic ligand-gated GABAA or GABAC receptors and G-protein-coupled GABAB receptors. Application of GABA in acute brain slices from young and adult mice significantly reduces the migration speed of RMS neuroblasts, an effect mediated by GABAA receptors (Bolteus and Bordey 2004). In contrast, pharmacological blockade of GABAA receptor activity increased migration rate, suggesting the presence of an endogenous GABAergic tone controlling neuroblast migration. Following high K+ application, neuroblasts release GABA, which can act in an autocrine-paracrine manner on their GABAA receptors and decrease migration speed by interfering with intracellular Ca2+ signaling independent of cell depolarization, most likely by affecting the release of Ca2+ from intracellular Ca2+ stores. Since GABA release is promoted by cell depolarization and GABA is able to depolarize neuroblasts, this would create a positive feedback loop causing further increase in extracellular GABA. Interestingly however, astrocytes surrounding the migratory neuroblasts are able to modulate GABA levels in the environment by controlling GABA uptake via the high-affinity GABA transporter subtype GAT4, which can be detected on the astrocytic processes ensheathing GABA-containing neuroblasts (Bolteus and Bordey 2004) (Fig. 9.8). Therefore, astrocytes help to maintain appropriate GABA levels in the microenvironment to ensure proper neuroblast motility.
Other neurotransmitter receptors progressively appear on neuroblasts during migration along the RMS, including the metabotropic glutamate receptor mGluR5, the Ca2+ permeable GluK5 kainate receptor and NMDA receptors (Platel et al. 2008b, 2010). Also in this case astrocytes surrounding the RMS seem to play a major role by releasing glutamate in a Ca2+–dependent manner to activate NMDA receptors on neuroblasts, thus providing an important in vivo survival cue for neuroblasts before their differentiation in the OB. However, NMDA activity does not seem to regulate neuroblast migration. Similarly, there is some evidence that mGluR5 promotes neuroblast survival, but has no effect on motility (Platel et al. 2008a). In contrast, pharmacological inhibition of GluK5 kainate receptor significantly promoted neuroblast migration in acute slices, suggesting that GluK5 receptors are tonically activated in migrating neuroblasts to decrease their speed, somehow cooperating with the action of GABA (Platel et al. 2008b). Additional experiments examining neural progenitor proliferation support the idea that glutamate and GABA provide a homeostatic system regulating neuroblast production and migration along the RMS. How the functions of these two neurotransmitters are coordinated in migrating neuroblasts is still not understood, and is made more complex by the heterogeneous expression of GABAA, GluK5 and mGluR5 in these cells (Platel et al. 2008a; Young et al. 2011) and by the ability of growth factors and other extracellular signals to regulate expression of multiple neurotransmitter transporters and receptors.
3.5 Growth Factors
Insulin-like Growth Factor-I (IGF-I): Several growth factors are present in the SVZ/RMS/OB system and their actions interplay to achieve optimal neural progenitor proliferation, neuroblast migration and differentiation. Igf-I −/− mice display impaired radial migration in the OB, with lower amounts of neuroblasts found in the glomerular layer and a parallel increase in neuroblast density at the exit of the RMS (Hurtado-Chong et al. 2009). In addition, a significant accumulation of DCX+ neuroblasts was observed in the SVZ, suggesting a defect in the ability of neuroblasts to leave the SVZ to initiate migration. Interestingly, while wild-type and Igf-I −/− neuroblasts migrate similarly in vitro, addition of IGF-I significantly stimulated migration of cells out of wild-type RMS explants, an effect that was blocked by PI3 kinase and Src kinase inhibitors. Based on these observations and on the fact that IGF-I is released by the choroid plexus of the lateral ventricle, IGF-I is likely to modulate neuroblast motility by influencing adhesion during migration. Besides promoting neuroblast exit from the SVZ, a second putative role for IGF in the OB would be to maintain adequate levels of phosphorylated Dab1, a scaffold protein operating downstream of Reelin to guarantee proper radial migration and positioning of differentiating interneurons.
Vascular Endothelial Growth Factor (VEGF): VEGF belongs to a family of glycoproteins playing a fundamental role in the development of blood vessels, angiogenesis and hematopoiesis. The VEGF family includes six different homologous factors (VEGF-A-E). VEGF-A binds to the receptor tyrosine kinases VEGF receptor-1 (VEGFR-1, or fms-related tyrosine kinase-1, Flt-1) and VEGF receptor-2 (VEGFR-2 or fetal liver kinase 1). A first study showed that SVZ-derived neural progenitors express both VEGFR-1 and -2 and that VEGF-A stimulated the migration of progenitors from SVZ explants by acting via VEGFR-2 (Zhang et al. 2003). A second report analyzing the phenotype of VEGFR-1 signaling deficient mice (Flt-1TK −/− ) revealed lower amounts of DCX+/BrdU+ cells in the RMS and a significant increase in the amount of BrdU+ cells found in the outer layers of the OB, consistent with a greater supply of newly formed cells to the OB compared to wild-type mice. Deleting Flt-1 specifically enhances the proliferation of neural progenitor cells in the SVZ, affects the cell type composition of the OB by promoting differentiation into dopaminergic olfactory neurons, but also promotes faster movement of neuroblasts, as indicated by in vitro migration assays and by a bigger OB size (Wittko et al. 2009). Interestingly, these effects are phenocopied by intracellular infusion of VEGF-A in wild-type mice, supporting the idea that deletion of VEGFR-1 signalling leads to increased amounts of VEGF-A protein in the SVZ/RMS, which in turn cause higher levels of phosphorylated VEGFR-2 in migratory neuroblasts. Intriguingly, VEGFR-2 phosphorylation is abolished when neuroblasts detach from the RMS and enter the OB, suggesting an important role for VEGF-A/VEGFR-2 in tangential migration. VEGF-A is expressed by glial cells in the SVZ and RMS, and in the same study VEGFR-1 also appears to be confined to GFAP+ astrocytes. While VEGF-A may have a paracrine role in regulating migration by acting on VEGFR-2 present in migratory neuroblasts, the potential roles of VEGF signaling on remodeling the astrocytic tunnels guiding neuroblasts towards the OB and the signaling pathways modulating VEGFR-2 activation at the RMS exit need further elucidation. In this regard, a recent study on the function of astrocyte-produced VEGF proposes that this factor may have an indirect role on neuroblast motility by modulating the vasculature used as a scaffold by migrating neuroblasts (Bozoyan et al. 2012). Therefore, a direct effect of VEGF on neuroblast migration along the RMS remains to be conclusively demonstrated.
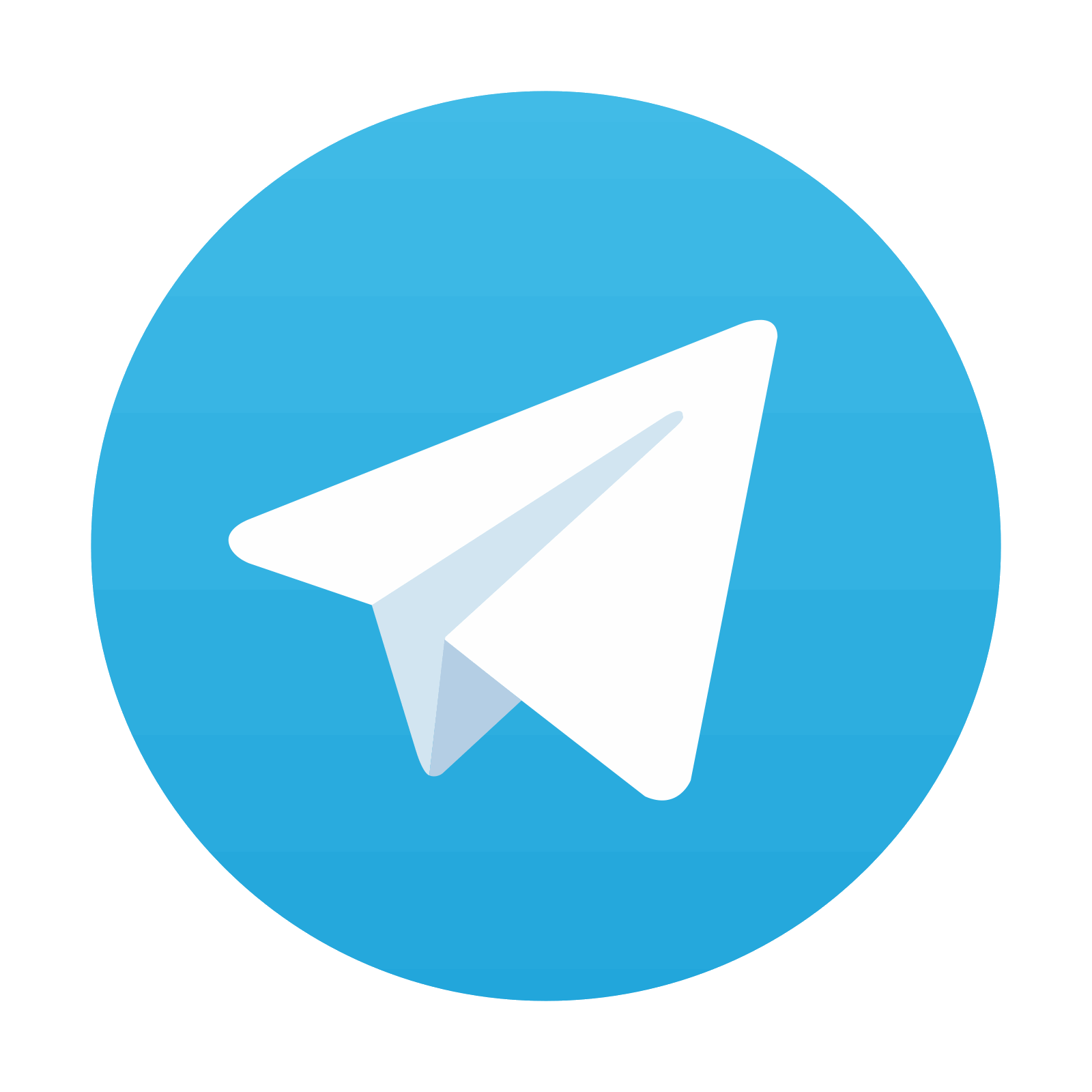
Stay updated, free articles. Join our Telegram channel
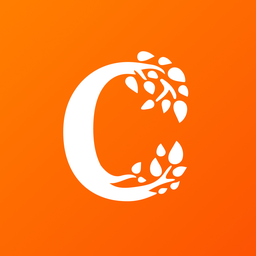
Full access? Get Clinical Tree
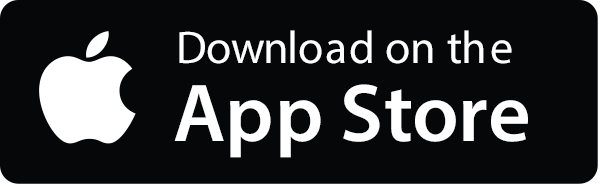
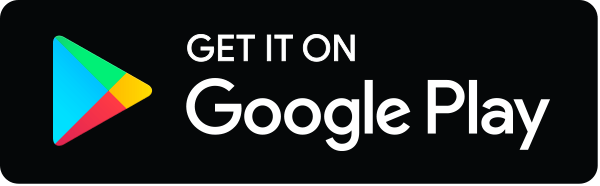