(1)
Facultad de Ciencias Médicas, Pontificia Universidad Católica Argentina, Buenos Aires, Capital Federal, Argentina
Abstract
The periphery of the autonomic nervous system (ANS) comprises two parts: the sympathetic and the parasympathetic systems. The identity and functional feature of the ANS innervating the gastrointestinal is often considered the third section of the ANS, i.e., the enteric ANS. This Chapter discusses the neurochemical bases of the peripheral motor and sensory components of the ANS, including the neurotransmitters and receptors involved and their participation in spinal autonomic reflexes. The composition and functions of the enteric nervous system are also discussed.
Keywords
Adrenergic neurotransmissionCholinergic neurotransmissionCo-transmissionEnteric nervous systemIonotropic transmissionMetabotropic transmissionParasympathetic nervous systemPrevertebral and paravertebral sympathetic gangliaSensory autonomic neuronsSpinal autonomic reflexesSympathetic nervous systemObjectives
After studying this chapter, you should be able to:
Underline why cholinergic and adrenergic neurotransmission and peptidergic co-transmission are the basis of the peripheral motor constituents of the autonomic nervous system (ANS).
Describe the location of the cell bodies and axonal trajectories of preganglionic and postganglionic sympathetic and parasympathetic neurons.
Name the neurotransmitters that are released by preganglionic autonomic neurons, postganglionic sympathetic neurons, postganglionic parasympathetic neurons, and adrenal medullary cells.
Name the types of receptors on autonomic ganglia and on various target organs and the processes involved in neurotransmission within the ANS.
Define sensory autonomic neurons, their anatomy, and how the basic sensorial dimensions are coded.
Describe the homologies and differences between spinal motor reflexes and spinal autonomic reflexes.
Describe the anatomical and physiological basis of genitourinary reflexes.
Describe the anatomical and physiological basis of defecation.
Describe the anatomical and physiological basis of pupillary reflexes.
Describe the composition and functions of the enteric nervous system.
Understand the meaning of local autonomic projections in neuroendocrine communication.
Define how the ANS contributes to the maintenance of healthy bone tissue.
The Organization of the ANS at a Peripheral Level Comprises Two Neurons in a Series
The periphery of the autonomic nervous system (ANS) comprises two parts: the sympathetic and the parasympathetic systems (Figs. 3.1 and 3.2). The identity and functional features of the ANS innervating the gastrointestinal is often considered the third section of the ANS, i.e., the enteric ANS. Conceptually, however, the regulatory mechanisms at the digestive level, although complex, do not escape the general characteristics of the sympathetic and parasympathetic systems discussed below [1].



Fig. 3.1
The sympathetic branch of the autonomic nervous system. Modified with permission from Cardinali [1]

Fig. 3.2
The parasympathetic branch of the autonomic nervous system. Modified with permission from Cardinali [1]
Moreover, the ANS has also been conceptualized as having five components: the sympathetic noradrenergic system, the sympathetic cholinergic system, the parasympathetic cholinergic system, the sympathetic adrenergic system, and the enteric nervous system [2]. The reason for the differential noradrenergic vs adrenergic responses is that sympathetic noradrenergic system activation dominates the responses to orthostasis, moderate exercise, and exposure to cold, whereas sympathetic adrenergic system activation dominates responses to glucoprivation and emotional distress [2].
Effectors of the sympathetic system are generally the smooth muscle, the heart, exocrine and endocrine glands, the adipose tissue, liver, lymphohematopoietic organs, and kidney. Most sympathetic ganglia are remotely located to the innervated organ, and therefore postganglionic axons (i.e., the axons of ganglion neurons) are long (Fig. 3.3). An exception is the short adrenergic neurons located on the wall of the genitourinary organs, which have a similar pattern to the parasympathetic array [3, 4].


Fig. 3.3
Adrenergic and cholinergic neurotransmission in the autonomic nervous system. Modified with permission from Cardinali [1]
Prevertebral ganglia (celiac, superior mesenteric, inferior mesenteric) give rise to postganglionic fibers, which through plexuses or nerves innervate organs of the abdominal and pelvic regions. The sympathetic preganglionic neurons have their bodies in the intermediolateral column of the spinal cord, between segments T1 and L2, so that the sympathetic system is also called thoracolumbar (Fig. 3.1). The preganglionic fibers exit through the corresponding anterior roots and follow the white communicating branches to the paravertebral sympathetic ganglion chain, from where four pathways can follow (Fig. 3.4) [3, 4]:

- 1.
To synapse with ganglion neurons of the corresponding level or higher or lower levels. The postganglionic fibers of these ganglion neurons leave the ganglion through the gray communicating branch and join the somatic peripheral nerves to innervate blood vessels, exocrine or endocrine glands, immune tissue, and piloerector muscles in their territories. The postganglionic fibers present a variable segmental distribution, much more poorly delimited than that of the sensory and motor fibers.
- 2.
The superior thoracic roots form synapses in the cervical ganglia, whose postganglionic fibers are distributed to innervate cranial structures (superior cervical ganglion, SCG) or to innervate thoracic organs via visceral, cardiac, and pulmonary nerves, by innervating these thoracic organs (middle cervical ganglion, stellate ganglion).
- 3.
The preganglionic fibers that emerge from the spinal roots below the diaphragm pass through the paravertebral sympathetic chain and they give rise to the splanchnic nerves, to end up forming synapses in the prevertebral ganglia. From these, the postganglionic fibers form small visceral nerves that, in the form of plexuses, are distributed to the abdominal and pelvic viscera.
- 4.
Finally, some preganglionic fibers also follow this path to form synapses directly with the chromaffin cells of the adrenal medulla, which represent the paravertebral sympathetic ganglia corresponding to the postganglionic cells.

Fig. 3.4
Neurons of the autonomic reflex , integrated at the level of the intermediolateral column of the spinal cord. The visceral afferents enter through the spinal nerve. The sympathetic and prevertebral ganglia and the gray and white communicating branches are also shown. Modified with permission from Cardinali [1]
These neurons form a bilateral chain parallel to the vertebral column, between the base of the skull and the coccyx. Normally, the anatomical distribution of each ganglionic chain consists of about 24 ganglia. In the cervical region, there are three ganglia, superior, middle, and inferior, although the latter is often fused with the first thoracic ganglion forming the stellate ganglion. In the other regions, there are usually a pair of ganglia for each spinal metamere, 10 or 11 thoracic ganglia, 4 lumbar, 3 or 4 sacral, and a single coccygeal.
The sympathetic prevertebral ganglia are located around the branches of the abdominal aorta, forming the abdominal or solar plexus. The main prevertebral ganglia are celiac, superior mesenteric, inferior mesenteric, and aortorenal.
The preganglionic neurons of the parasympathetic system have their perikarya in nuclei of the brainstem or in the lateral column of the sacral spinal cord, which is why it receives the designation of craniosacral division (Fig. 3.2).
In the cranial portion the preganglionic neurons are in the visceral efferent nuclei, from where they distribute their fibers peripherally via the cranial nerves [5]:
Edinger–Westphal nucleus: common ocular motor nerve (III).
Superior and lacrimal salivary nucleus: facial nerve (VII).
Lower salivary nucleus: glossopharyngeal nerve (IX).
Dorsal nucleus of the vagus and ambiguous nucleus: vagus nerve (X).
These fibers are distributed to terminal ganglia, close to the effector organs, from which short postganglionic fibers are formed, giving rise to the innervation of the viscera of the head and neck, the thoracic cavity, and much of the abdominal cavity.
On the other hand, the preganglionic neurons of the sacral portion of the spinal cord, located in the intermediolateral columns between the S2 and S4 segments, send their fibers through the anterior roots to form the pelvic nerves and end in the terminal ganglia of the pelvic plexus, innervating the descending colon and the urogenital organs [6].
The parasympathetic terminal ganglia are formed by small clusters of neurons, located on or in the walls of the viscera, where the preganglionic fibers form synapses. In the cephalic region, there are four relatively large ganglia, associated with branches of the trigeminal nerve: the ciliary, sphenopalatine, optic, and submandibular ganglia.
In the cervical, thoracic, and pelvic regions, there are small ganglia that form visceral plexuses, whereas in the digestive tract they are in the myenteric plexus of Auerbach and the submucosal plexus of Meissner.
The cell bodies of the parasympathetic preganglionic neurons are located in the brainstem and sacral cord. Although some of their axons are myelinated, most are unmyelinated. Compared with the sympathetic system, the preganglionic axons are longer and their postganglionic fibers considerably shorter. This is because the parasympathetic ganglia are located in the immediate vicinity of the innervated organs [1].
Effectors of the parasympathetic system include smooth muscle and glands of the digestive tract, the excretory organs, the genital system, heart, and lung, lymphohematopoietic and endocrine organs and intraocular muscles. Except genital arteries and possibly the brain, there is no parasympathetic innervation of vascular smooth muscle. Nor is there a parasympathetic innervation of the skin [1]. By using different experimental models of autonomic hyper- or hypofunction, the conclusions listed in Table 3.1 can be reached [7].
Table 3.1
Autonomic nervous system effects on effector organs
Organ, tissue | Parasympathetic stimulation | Sympathetic stimulation |
---|---|---|
Pupils | ||
Radial muscle of the iris | – | Contraction, α1 adrenoceptor mediated |
Sphincter muscle of the iris | Contraction, M3, M2-receptor mediated | – |
Ciliary muscle | Contraction, M3, M2-receptor mediated | Weak relaxation, β2 receptor mediated |
Tracheobronchial muscle | Contraction, M2, M3-receptor mediated | Relaxation, β2 adrenoceptor mediated |
Heart | Bradycardia, negative inotropism, M2 receptor mediated | Tachycardia, positive inotropism, β1 adrenoceptor mediated |
Arteries | ||
Skin, mucosas | – | Constriction, α1 adrenoceptor mediated |
Abdomen | – | Constriction, α1 adrenoceptor mediated Dilatation (blood epinephrine), β2 adrenoceptor mediated |
Skeletal muscle | – | Dilation, M2 receptor mediated Dilatation (blood epinephrine), β2 adrenoceptor mediated |
Coronary arteries | – | Constriction, α1 adrenoceptor mediated Dilation, β2 adrenoceptor mediated |
CNS arteries | Dilation (?) | Constriction, α1 adrenoceptor mediated |
Penis | Dilation, M2 receptor mediated | – |
Kidney | – | Constriction, α1 adrenoceptor mediated |
Veins | – | Constriction, α1 adrenoceptor mediated |
Endothelium | Stimulation of NO synthase, M3 receptor mediated | |
Bladder | ||
Detrusor muscle | Contraction, M3 receptor mediated | Relaxation, β2 adrenoceptor mediated |
Internal sphincter | Relaxation, M2 receptor mediated | Constriction, α1 adrenoceptor mediated |
Genital tract | ||
Seminal vesicles | – | Constriction, α1 adrenoceptor mediated |
Vas deferens | – | Constriction, α1 adrenoceptor mediated |
Intercourse | Erection, M3 receptor mediated | Ejaculation, α-1 adrenoceptor mediated |
Uterus | – | Constriction, α1 adrenoceptor mediated Relaxation, β2 adrenoceptor mediated |
Gastrointestinal tract | ||
Circular and longitudinal muscle | Contraction, M3 receptor mediated | Relaxation (direct) β adrenoceptor mediated Parasympathetic inhibition, α1 adrenoceptor mediated |
Sphincters | Relaxation | Constriction, α adrenoceptor mediated |
Exocrine glands | ||
Salivary | Secretion. M3 receptor mediated | |
Lacrimal | Secretion. M3 receptor mediated | – |
Digestive | Secretion. M3 receptor mediated | Inhibition, α adrenoceptor mediated |
Nasopharyngeal | Secretion. M3 receptor mediated | – |
Sweat | – | Secretion. M3 receptor mediated |
Bronchial | Secretion. M3 receptor mediated | Weak inhibition, α-1 adrenoceptor mediated. Weak stimulation, β adrenoceptor mediated |
Adipose tissue | – | Lipolysis, β1 adrenoceptor mediated |
Immune system | ||
Lymph nodes, spleen | Stimulation | Inhibition, α-1 adrenoceptor mediated |
Splenic capsule | – | Constriction, α-1 adrenoceptor mediated |
Endocrine system | ||
Juxtaglomerular apparatus | – | Renin secretion, β1 adrenoceptor mediated |
Thyroid follicles | Thyroid growth, secretion of T4. M receptor mediated | Inhibition of T4 secretion, α1 mediated. Weak stimulus of T4 secretion, β adrenoceptor mediated |
Thyroid C cells | Inhibition of CT secretion | Inhibition of CT secretion, α1 mediated. Weak stimulus of CT secretion, β adrenoceptor mediated |
Parathyroid glands | Inhibition of PTH secretion | Inhibition of PTH secretion |
Pineal gland | – | Melatonin release, β2 adrenoceptor mediated. Weak stimulus of melatonin release, α1 adrenoceptor mediated |
Adrenal medulla | Preganglionic stimulation, M1 receptor mediated | |
Anterior pituitary | Postganglionic SCG sympathetic neurons inhibit FSH, LH, GH, PRL, and TSH secretion and augments ACTH secretion | |
Posterior hypophysis | – | Postganglionic SCG sympathetic neurons inhibit ADH secretion, β adrenoceptor mediated |
Exocrine pancreatic glands | Secretion. M1 receptor mediated | Inhibition, α adrenoceptor mediated |
Pancreatic islets, α cells | Secretion. M1 receptor mediated | Inhibition of glucagon secretion, α2 adrenoceptor mediated. Weak stimulation of glucagon secretion, β2 adrenoceptor mediated |
Pancreatic islets, β cells | Secretion. M1 receptor mediated | Inhibition of insulin secretion, α-2 adrenoceptor mediated. Weak stimulation of insulin secretion, β2 adrenoceptor mediated |
Liver | Glycogen synthesis (?) | Glycogenolysis, α1 adrenoceptor mediated Gluconeogenesis, β2 adrenoceptor mediated Lipolysis, β1 adrenoceptor mediated |
Cholinergic and Adrenergic Neurotransmission and Peptidergic Co-transmission Are the Basis of the Peripheral Motor Constituents of the ANS
Transmitters identified, partially or totally, in neural pathways comprise five large families [1]:
Biogenic amines: norepinehrine (NE), epinephrine (E), acetylcholine (ACh), 5-HT, dopamine (DA), His
Amino acids: Glu, aspartate, GABA, Glycine (Gly), taurine and purine derivatives (adenosine, ATP, included in this group although they are not amino acids).
Neuropeptides (over 70 different structures identified to date)
Gases, such as NO or CO (carbon monoxide)
Lipids, such as anandamide.
The criteria for a substance to be considered a neurotransmitter are as follows (Fig. 3.5):

It must be synthesized by the presynaptic neuron and stored in synaptic vesicles (although exceptions are found, like the gases and lipids)
The physiological neural stimulation must release it
It must act on the postsynapsis in a similar manner to normal stimulus analyzed (action identity criterion)
There should be effective mechanisms for terminating its action (reuptake in neural terminal, metabolism, passage to extrasynaptic space), to ensure the necessary speed and transience of transmitter action

Fig. 3.5
Chemical synapse. (a) In the resting synapse the pre- and postsynaptic membranes are normally polarized. (b) In the active synapse, depolarization of the neural terminal (secretory potential) results in the release of the transmitter, which diffuses through the synaptic gap and produces local synaptic currents and potentials in the postsynaptic membrane, which initiate effector activity, neuronal transmission, neurotransmitter release, hormonal secretion, muscle contraction). Modified with permission from Cardinali [1]
Among them, the action identity criterion is the most important, as it involves the physiological effect itself [1].
Listed criteria have been completely fulfilled in the peripheral ANS, for the neurotransmitter nature of ACh or NE (various preganglionic and postganglionic territories). On the other hand, in central ANS neurons, the physiological characterization of neurotransmitters has been difficult, owing to the large number of synapses present, to the neuronal plurality of most brain regions, and to the very common coexistence of two or more neurotransmitters in the same synapse.
Biogenic amines participate in approximately 5% of the brain synapses, localizing in certain subcortical projection pathways to encephalic or descending spinal cord regions. The noradrenergic and serotonergic cortical, cerebellar, and subcortical innervation originates, almost exclusively, in brainstem nuclei that project in the form of a “spider web” to large cerebral areas, an anatomical disposition that speaks of its general modulatory function (Chap. 1). Something similar occurs for the central dopaminergic, cholinergic, and histaminergic systems. It is not surprising, then, that these monoaminergic systems have been linked to generalized alterations in brain function, such as psychiatric illness, emotionality, wakefulness, and sleep (Chap. 4).
In the ANS, ACh is the transmitter of all the preganglionic synapses, of all parasympathetic postganglionic neurons, and of some sympathetic postganglionic neurons (muscular vasodilator system, sweat glands). NE is the neurotransmitter of all the remaining postganglionic sympathetic neurons [3, 4].
For most chemical synapses, there is an exocytotic release from the presynapsis of the neurotransmitter substance contained in the synaptic vesicles. Exceptions to this rule are gases identified as neurotransmitters (NO, CO), which pass through membranes by simple diffusion, and neurotransmitter lipids such as anandamide, which are not stored in the vesicles.
In the case of NO, which is one of the most important local regulators of BP, three different NO synthase (NOS) isoforms have been described, two constitutively present (eNOS and nNOS), and one inducible (iNOS). In the CNS, both eNOS and nNOS are present. Centrally, NO functions mainly as a neuromodulator, having both sympathoinhibitory and sympathoexcitatory actions [8]. At the periphery, locally released NO from endothelial cells acts on adjacent vascular smooth muscle cells to produce vasodilatation. In addition, NO synthase activity has been demonstrated in preganglionic autonomic fibers innervating vascular smooth muscle. Furthermore, NO release from autonomic nitrergic nerves interferes with the release of NE. Both central and peripheral effects of NO under normal conditions are masked by the baroreflex [9] (Chap. 4).
In general, the action of these signals is exerted at the level of specific receptors in the postsynapsis. The gases are again the exception as they traverse the postsynaptic membrane and exert their action intracellularly. In the chemical synapse the synaptic message is unidirectional (it ranges from pre- to postsynapsis) and when it comes from synapses by exocytotic release of neurotransmitters present in vesicles, it shows a synaptic delay. This synaptic delay is largely due to the transmitter release process, and to a minimal extent by the passage of the transmitter through the synaptic gap. Its duration is about 0.5–1.0 ms [10].
In the ANS, the synaptic cleft is broad (synaptic varicosities or nondirected synapses) and thus differs from directed synapses, such as the neuromuscular plaque (Fig. 3.6). This allows the “transmission by volume” into the ANS, a name that is given to the wide diffusion of the autonomic transmitter to several postsynaptic cells.


Fig. 3.6
Postganglionic noradrenergic neuron with its distribution in varicosities (“beads”). Modified with permission from Cardinali [1]
Autonomic postganglionic fibers present a series of synaptic dilations or varicosities when they approach their targets, which have a length of approximately 1 μm and a diameter of between 0.5 and 2 μm. These varicosities contain a high density of mitochondria and synaptic vesicles. The synaptic space has a variable width; for example, in the innervation of the smooth muscle, it varies from 20 nm in the vas deferens to 1–2 μm in the large arteries. Transmitter release occurs in a passage, and propagates nerve impulses along the autonomous axon. The availability of varicosities and the wide synaptic space allow the released neurotransmitter to diffuse variable distances in the target organ and to activate multiple receptors, which expand the effect of autonomic activity (Fig. 3.6) [3, 4, 11].
This classic view of autonomic transmission is, however, a simplification, as it is now known that many of these neurons also use other types of molecules as neurotransmitters or as neuromodulators. Initially, these were encompassed under the denomination of non-adrenergic–noncholinergic transmission, which mainly includes purinergic transmitters and neuropeptides. Purinergic nucleotides , such as ATP, are important transmitters in nerve fibers that innervate the intestinal smooth muscle, the urinary bladder, and the vas deferens, where they act directly as neurotransmitters or modulate the effects of NE or ACh. Several neuropeptides have been identified, widely distributed, in autonomic ganglia, the enteric system, and peripheral fibers. The most well-known include: enkephalin/endorphin, vasoactive intestinal peptide (VIP), substance P, calcitonin gene-related peptide (CGRP), neuropeptide-Y (NPY), somatostatin, bombesin, galanin, neurotensin, angiotensin, and cholecystokinin (CCK)/gastrin.
The coexistence of neuropeptides with classical transmitters in different autonomic neurons is the rule, for example, sympathetic and parasympathetic postganglionic neurons containing ACh and VIP, or adrenergic neurons containing NE and NPY. The classical neurotransmitter and the neuropeptide may be separately released under different excitation conditions (Fig. 3.7). It is postulated that neuropeptides might act as transmitters by themselves or as neuromodulators, altering the action of classical transmitters. Thus, stimulation of cholinergic fibers from the salivary or sweat glands causes release of ACh, which has a secretory effect, and VIP, which produces vasodilation [3].


Fig. 3.7
Differential release of transmitters contained in small dense-cored vesicles (biogenic amine) and large dense-cored vesicles (neuropeptide). Modified with permission from Cardinali [1]
The neuropeptides co-released with small molecule neurotransmitters in autonomic nerves do not usually act as co-transmitters, but rather as prejunctional neuromodulators or trophic factors. Autonomic co-transmission offers subtle, local variation in physiological control mechanisms, rather than the dominance of inflexible central control mechanisms envisaged earlier [12]. The variety of information imparted by a single neuron then greatly increases the sophistication and complexity of local control mechanisms. Co-transmitter composition shows considerable plasticity in development and aging, in pathophysiological conditions, and following trauma or surgery. For example, ATP appears to become a more prominent co-transmitter in inflammatory and stress conditions [12]. In situations such as congestive cardiac failure that are characterized by high levels of cardiac sympathetic drive, sympathetic co-transmitters such as NPY can be released in addition to NE. Even in the presence of β-adrenoceptor blockers, NPY is able to bind to its own receptors located on the cholinergic ganglia and ventricular myocytes, thus inhibiting ACh release during vagus nerve stimulation and limiting the subsequent bradycardia [13].
Most experimental evidence has shown that the autonomic sympathetic and parasympathetic control is organized in different functional units or groups of neurons that innervate specific target organs. Thus, in the sympathetic ganglia, subtypes of vasomotor, sudomotor, pilomotor, and visceromotor neurons are distinguished. Ganglion neurons of each subtype present a unique chemical code (Table 3.2). Today, we know that the rule is that the ANS releases a combination of neurotransmitters whose proportion depends on the intensity and frequency of stimulation (Fig. 3.7).
Table 3.2
Neurochemical code in different types of autonomic nerve fibers
Nerve fibers | Neurotransmitters |
---|---|
Vasoconstriction | NE, NPY |
Vasodilation | ACh, VIP |
Sudomotor | ACh, VIP, CGRP |
Piloerector | NE, dynorphin |
Sialomotor | CGRP, NPY |
Intestinal ganglia | NE, somatostatin |
It is useful to pay attention to a differential aspect among the different families of neurotransmitters, namely, the mechanism of synthesis [1]. Biogenic amines, amino acids, gases, and lipids are synthesized by an enzymatic process at the synaptic terminals. The specific enzymes migrate to the terminal via axoplasmic transport, forming parts of vesicles, and catalyze at the terminal the synthesis of transmitter from specific precursors. Because of its catalytic nature, an enzyme molecule participates in the synthesis of thousands of molecules of the transmitter. This prevents the rapid reduction of the contents of the transmitter.
In contrast, neuropeptides are synthesized in the neuronal body as part of a higher molecular weight prepropeptide, which is incorporated into the synaptic vesicles and is processed (by acetylation, glycosylation, or hydrolysis reactions)while these vesicles migrate by axonal transport toward the neural terminal. It should be noted that both the axon and the presynaptic terminals are almost devoid of ribosomes, and hence of the ability to synthesize peptides or proteins (there is only a restricted synthesis of some components of the cytoskeleton). In this, they differ from the dendrites [3].
It is for this reason that after a prolonged neuronal stimulation there is a greater possibility of neuropeptide transmitter depletion than that of any co-transmitter (Fig. 3.7). The peptide transmitter deposits are depleted at terminals remote from the neuronal body, the terminals at close range being maintained for longer. This is the reason why, on certain occasions, terminals of the same neuron can release different combinations of transmitting substances [12]. Likewise, the coexistence of neurotransmitters does not mean that they are released with the same kinetics before presynaptic stimulation. As shown in Fig. 3.7, small vesicles with a dense center with a biogenic amine (e.g., NE) are released before the large vesicles (e.g., NPY).
Generally, the opening or closing of channels at the postsynaptic membrane by neurotransmitter in the autonomic synapses (Fig. 3.8) is produced by: (a) the direct association of neurotransmitter with the postsynaptic receptor coupled to a channel (ionotropic transmission); (b) by synthesizing intracellular second messengers, triggered by the association of the autonomic transmitter with its receptor, this second messenger being responsible for the modification of membrane conductance (metabotropic transmission) [7].


Fig. 3.8
Ionotropic and metabotropic effect of neurotransmitters. Modified with permission from Cardinali [1]
An example of ionotropic transmission is the transmission given by the nicotinic cholinergic receptor of the autonomic preganglionic synapse (Fig. 3.9). The receptor forms a constituent part of an ion channel that permeates Na+ and to a lesser extent K+, and that opens when ACh binds to the receptor.
An example of metabotropic transmission is the β action of NE, which occurs by increasing cAMP and the subsequent phosphorylation of K+ channels to inactivate them. The result of this action is the postsynaptic depolarization that follows the increases in the concentration of intracellular K+ (Fig. 3.10).


Fig. 3.10
Sequence of events produced by the interaction of NE with β-adrenoceptors. See text. Modified with permission from Cardinali [1]
Autonomic ionotropic transmission is rapid, whereas metabotropic transmission is slower. This fact is due to the time of synthesis and intracellular translocation of the second messenger synthesized from the cell membrane to its site of action.
The molecular biological studies on various neurotransmitter receptors indicate the existence of two structural superfamilies, which correspond to the two types of transmission (ionotropic and metabotropic) analyzed above [7]:
Receptors associated with ionophores, whose quaternary structure is part of an ion channel. This is the case of the nicotinic cholinergic receptor, which comprises the following subtypes: muscle, ganglion, neuronal, neuronal CNS, neuronal α7 (more recently they have been molecularly defined as α1*-, α2*-, α3*-, α4*-, α6*-, α7*-, α8*-, and α9*-acceptors, nACh). This is also the case for the GABA receptor types A and C, the glycinergic receptor, the AMPA, kainate and N-methyl-d-aspartate (NMDA) receptors for Glu, the serotonergic receptor 5-HT3 subtype, and the ionotropic purinergic receptors. The structure consists of highly homologous protein sequence subunits that derive from a common gene. Each of the subunits contains 15–20 amino acid hydrophobic regions that constitute alpha-helices, which completely cross the plasma membrane.
Receptors associated with G-proteins : the receptor structure constituting a single subunit with seven hydrophobic protein portions spanning the cell membrane. Each of, the α-helices has 15–20 amino acids, which are not arranged in the form of an ionic channel as in the previous case. The ion channel is located distant to the receptor and is not part of it; it can instead be used by several receptors (Fig. 3.10).
This is a very large family of receptors and comprises, among others, α1 adrenergic receptors (whose subtypes are identified as α1A, α1B, and α1D), α2 (subtypes α2A, α2B, α2C), β (subtypes β1, β2, β3, and β4); muscarinic cholinergic receptors (subtypes: M1, M2, M3, M4, and M5); dopaminergic receptors (subtypes D1, D2, D3, D4, and D5); serotonergic (5-HT1A, 5-HT1A, 5-HT2A, 5-HT2B, 5-HT2C, 5-HT4A, 5-HT4A, 5-HT5A, 5-HT6, 5-HT7) subtypes; histaminergic (subtypes H1, H2, H3, H4); metabotropic Glu receptors (mGlu1 to mGlu8 subtypes), GABA type B receptor, melatonin receptor (MT1 MT2 subtypes), conopsins, and receptors of different types of neuropeptides.
There is intense “cross-talking” between the second messenger pathways, as in many cases individual enzymes, channels or cytoskeletal proteins can be modified at more than one site of the molecule by different systems of second intracellular messengers. It is estimated that the metabotropic pathway is about 10,000 times slower than the ionotropic pathway [11].
The activation of G proteins does not always lead to the synthesis of a second messenger mediating the effect. Effects of the G proteins are directly exerted on channels, without the participation of the phosphorylation. For example, the hyperpolarization produced by ACh in the heart is caused, in a first-stage effect, by a G protein that opens K+ channels without the mediation of a second messenger. There are other similar actions described on Ca2+ channels. Finally, there are situations in which the channel is quickly affected by the G protein, and much later modified by the second messenger triggered by the same G protein (this is the case of the muscarinic M2 receptor type in the heart) [7].
In addition to the early and rapid effects of autonomic neurotransmitters, there are other late effects on neuronal function. In general, the action of the transmitter triggers modifications in gene expression, with lasting changes in cellular function until long after the synaptic action is over. One of the first known late effects was the trans-synaptic induction of tyrosine hydroxylase, the limiting enzyme in NE synthesis, in postganglionic sympathetic neurons because of nicotinic presynaptic ganglionic activation.
Regarding ACh synthesis, it comprises the acetylation of choline catalyzed by the enzyme choline acetyltransferase. ACh metabolism is accomplished in the synaptic gap and involves the reversal of the synthesis reaction, i.e., a hydrolysis catalyzed by the enzyme acetylcholinesterase. In muscarinic cholinergic synapses this hydrolysis is rapid enough to ensure complete inhibition of the synaptic effect of ACh, a fact that does not occur in nicotinic synapses, such as the preganglionic one.
There are two types of receptors for ACh : muscarinic and nicotinic.
Muscarinic cholinergic receptors mediate the effects of ACh in postganglionic synapses on smooth muscle, endocrine or exocrine glands, immune cells, and the heart. They also mediate some of the effects of ACh in the CNS and autonomic ganglia. Five types of muscarinic receptors (associated with G protein) are known to exist [11]:
M1: associated with a decrease in K+ conductance (and therefore excitatory), present in the cerebral cortex and autonomic ganglia.
M2: associated with increased K+ conductance (and therefore it is inhibitory), present in the heart, and presynaptically on several parasympathetic territories.
M3 and M5: similar to M1, they are present in smooth muscle and glandular cells.
M4: similar to M2.
As in the case of other G protein-associated receptors, the action of cholinergic agonists can be exerted through two mechanisms, one quick, direct, via the protein G effect on the channel, and a slower one through the second intracellular messenger. M1, M3, and M5 receptors mediate cholinergic responses accompanying postsynaptic stimulation such as smooth muscle contraction of the bronchi, bowel, or bladder. M2 and M4 receptors mediate inhibitory responses, bradycardia, and negative inotropism verifiable in the heart after vagal stimulation or inhibition of presynaptic transmitter release.
Nicotinic cholinergic receptors are in the autonomic ganglia, CNS, and muscle plate. In all cases, they are associated with ionotropic responses involving the opening of Na + channels (which, although to a lesser extent, also permeate K+), and consequently depolarization (Fig. 3.9). These channels are composed by pentamers resulting from combinations of 17 subunits. Nicotinic receptors are broadly classified into two subtypes based on their primary sites of expression: muscle-type nicotinic receptors and neuronal-type nicotinic receptors. In both muscle-type and neuronal-type receptors, the subunits are somewhat similar, especially in the hydrophobic regions. In the autonomic ganglia, hexamethonium is the specific nicotinic blocker, whereas in the muscle plate, curare (or its active ingredient, tubocurarine) is the blocker [7, 11].
In relation to NE , their synthesis is started by tyrosine hydroxylation to l-dopa (l-dihydroxyphenylalanine) catalyzed by the enzyme tyrosine hydroxylase (Fig. 3.11). This is followed by the decarboxylation of levodopa to DA and by β hydroxylation of DA to NE. The limiting step in this sequence is the first one, i.e., the hydroxylation of tyrosine (Fig. 3.11).


Fig. 3.11
Norepinephrine (NE) synthesi s. Tyrosine is converted to l-DOPA by tyrosine hydroxylase. DOPA is then decarboxylated to DA by l-aromatic amino acid decarboxylase. Finally, DA is β-hydroxylated to NE by DA β-hydroxylase. NE is metabolized by either monoamine oxidase (MAO) or catechol-O-methyltransferase (COMT)
Catabolism of NE is carried out by oxidative deamination by the action of monoamine oxidase enzyme (MAO), or by O methylation enzyme by catechol-O-methyl transferase (COMT; Fig. 3.12). However, under normal conditions, the most widespread form of termination of the action of NE is the presynaptic reuptake in an intact, unmetabolized form. The time at which this reuptake mechanism becomes saturated, metabolism by MAO or COMT ensues.


Fig. 3.12
Metabolism of NE by monoamine oxidase (MAO) and catechol-O-methyltransferase (COMT). Modified with permission from Cardinali [1]
There are two main types of adrenergic receptors (or adrenoceptors): (a) α-adrenoceptors; (b) β-adrenoceptors.
As in the case of ACh, these receptor types were identified using adrenergic agonists and antagonists, and more recently, various subtypes have been cloned and identified. They belong to the superfamily of receptors associated with G proteins.
The α-adrenergic effect is one that shows the following sequence of activity for agonists: NE = E > > isoproterenol (a synthetic adrenergic agonist).
The β-adrenergic effect is one that shows the following sequence of activity for agonists: isoproterenol > E = NE.
In turn, each type of adrenoceptor is subdivided into α1 (whose subtypes identified are α1A, α1B, and α1D), α2 (subtypes α2A, α2B, and α2C), and β1, β2, β3, and β4 [7, 11].
The subcellular mechanism of action of the α1 adrenoceptor is the increase in conductance to Ca2+ and the activation of the turnover of inositol phospholipids. The subcellular mechanism of α2 adrenoceptor is the inhibition of adenylate cyclase. The subcellular mechanism of action of β1, β2, β3, and β4 receptors is the activation of adenylate cyclase; α2 adrenergic receptors were initially described as having a presynaptic location and with negative modulation function of NE release. However, in the CNS, there are also α2 adrenoceptors at a postsynaptic location. The β1, β2, β3, and β4 adrenergic receptors have both pre- and postsynaptic localization.
The postsynaptic consequence of the mentioned autonomic ionotropic or metabotropic mechanisms is:
Depolarization, which can be reached by a spatial or temporal sum to trigger an action potential. The depolarizing synaptic potential is called the excitatory postsynaptic potential, or EPSP (Fig. 1.8).Stay updated, free articles. Join our Telegram channel
Full access? Get Clinical Tree
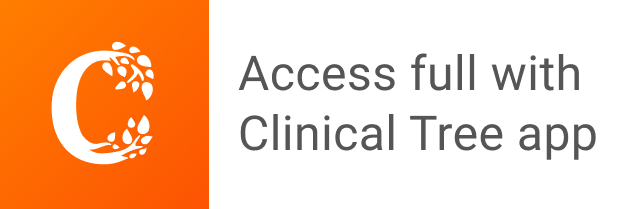