(1)
Facultad de Ciencias Médicas, Pontificia Universidad Católica Argentina, Buenos Aires, Capital Federal, Argentina
Abstract
The hypothalamus is a phylogenetically ancient part of the CNS, and its structure has remained relatively constant in terrestrial vertebrates throughout evolution. The hypothalamus participates as the third level of the ANS hierarchy in the coordination of specific behaviors (defense behavior, thermoregulation, feeding, sexual and maternal behavior). This Chapter discusses the hypothalamic mechanisms that control hormone secretion and how they vary during a 24-h cycle. The nature of the defense behavior, feeding behavior and temperature regulation is described.
Keywords
AllostasisBody temperature regulationBrown adipose tissueConstitutive and reactive secretion of hormonesDefense behaviorFeeding behaviorHydroelectrolytic balanceHypothalamic nuclei and areasHypophysiotropic areaSexual and maternal behaviorObjectives
After studying this chapter, you should be able to:
Name the areas and principal nuclei of the hypothalamus and describe how the hypothalamus participates as the third level of the ANS hierarchy in the coordination of specific behaviors
Describe the neural mechanisms that control the constitutive and reactive secretion of hypophysiotropic, adenohypophyseal, pineal, thyroid, and parathyroid hormones, and how they vary in the three body configurations (wakefulness, NREM sleep, REM sleep) during a 24-h cycle.
Describe the nature of the defense behavior as a paradigm of reactive homeostasis and differentiate homeostasis from allostasis.
Identify the principal physiological components of the feeding behavior and the location and function of the participating neurotransmitters and hormones. Describe how they vary in the three body configurations (wakefulness, NREM sleep, REM sleep) during a 24-h cycle.
Describe the neural and hormonal mechanisms that control 24-h rhythms in plasma osmolality and the intravascular volume by modifying water and electrolyte intake behavior.
Understand how the SON–PVN–neurohypophyseal system, the renin–angiotensin system, and the secretion of ANP vary in the three body configurations (wakefulness, NREM sleep, REM sleep) during a 24-h cycle.
Identify the homeostatic components of body temperature regulation and the location and function of the participating neural and hormonal mechanisms of shivering and nonshivering thermogenesis in the three body configurations (wakefulness, NREM sleep, REM sleep) during a 24-h cycle.
Name the hypothalamic basis of sexual and maternal behavior
Hypothalamic Behaviors Comprise Coordinated Mechanisms, Including Autonomic, Neuroendocrine, and Motivational Components
The hypothalamus is a phylogenetically ancient part of the CNS, and its structure has remained relatively constant in terrestrial vertebrates throughout evolution, unlike other brain regions, such as the neocortex or limbic system, which show marked interspecies differences.
The hypothalamus is the main governing center for homeostatic functions. A decerebrate animal, in which the brainstem is cut between the upper and lower colliculi, keeps the regulation of its internal environment intact, as the cut leaves the hypothalamus intact. In contrast, an animal with hypothalamic lesions requires extreme care to survive, as its homeostatic functions are greatly diminished or suppressed [1].
The hypothalamus is organized to perform autonomic, endocrine, and somatic functions. To do this, the region connects with the various components of the autonomic motor hierarchy, and with the somatosensory, motor, endocrine, and immune systems.
The principal hypothalamic nuclei are depicted in Figs. 5.1 and 5.2. In humans, the hypothalamus weighs about 5–8 g. Its anatomical limits are rather diffuse. As a ventral part of the diencephalon, the hypothalamus borders the third ventricle, below the thalamus. Caudally, it limits with the mesencephalon and rostrally, with the lamina terminalis, anterior commissure, and optic chiasm. Lateral to the hypothalamus the optic tract, the internal capsule, and the subthalamic structures are found.


Fig. 5.2
Sagittal view of the hypothalamus and at the level of the indicated cuts (optic chiasm and median eminence, ME). MPOA medial preoptic area, PVN paraventricular nucleus, DMN dorsomedial nucleus, AHA anterior hypothalamic area, VMN ventromedial nucleus, SCN suprachiasmatic nucleus, SON supraoptic nucleus, ARC arcuate nucleus, LHA lateral hypothalamic area
From a functional point of view, the hypothalamus should be considered part of a continuous neuronal component that extends from the midbrain to the basal regions of the telencephalon (limbic cortex). Together, this portion of the brain is anatomically linked to the old olfactory, limbic system by the medial forebrain bundle.
The hypothalamus comprises three zones (Fig. 5.2):
The periventricular area is a thin layer of nerve tissue adjacent to the third ventricle.
In the medial zone, there are several nuclei. The hypophysiotropic area is the portion of the medial zone participating in adenohypophyseal regulation. In the supraoptic nucleus (SON) and paraventricular nucleus (PVN) , the perikarya of the fibers forming the hypothalamic–neurohypophyseal tract and secreting arginine vasopressin (AVP) and oxytocin are located.
The lateral hypothalamic area (LHA) contains no distinguishable nuclei, but instead Golgi type II neurons , which surround the medial forebrain bundle. This bundle is continued rostrally with the basolateral structures of the limbic system and caudally with the rostral structures of the brainstem. The medial forebrain bundle is one of the largest CNS fiber bundles, which interconnects forebrain structures with brainstem structures, forming an extensive network profile.
The LHA is an area of greatest interconnectivity of the hypothalamus, allowing the modulation of various functions, from cognitive to autonomic. The LHA , also called interstitial nucleus of the medial forebrain bundle, is one of the regions of the CNS with maximal biochemical and cellular heterogeneity (more than 35 types of neurons described) [2].
The afferent and efferent connections of the hypothalamus indicate that it is an important center of integration for autonomic, somatic, and neuroendocrine functions. In general, LHA is reciprocally connected with the upper portion of the brainstem and upper limbic structures. It also receives somatic, inter- and exteroceptive impulses through the thalamus, cerebellum, and limbic system.
The medial hypothalamus has abundant reciprocal connections to the lateral hypothalamus, but receives few projections from other brain areas. Its function is mainly neuroendocrine. It contains receptors for humoral signals from the internal environment (glucose and other metabolites, temperature, osmolality, various hormones), its efferences being neuroendocrine (hypophysiotropic and neurohypophyseal peptides).
From a functional point of view, the hypothalamus is the level of the autonomic hierarchy that provides the complex program of the various homeostatic reactions, with their autonomic, neuroendocrine, and behavioral components. To achieve this, the hypothalamus uses the various elementary, segmental (medullary level) or system (brainstem level) programs, contained at lower levels of the autonomic hierarchy.
There are four hypothalamic functions: (a) neuroendocrine function; (b) regulation of the ANS; (c) hypothalamic behavior regulation; (d) control of biological rhythms [1].
The neuroendocrine function is mediated by the SON/PVN neurohypophyseal systems and by the hypophysiotropic area (Fig. 5.3).


Fig. 5.3
Neuroendocrine connections of the medial hypothalamus. Hypothalamic hypophysiotropic hormones ① reach the adenohypophysis through the pituitary portal system ② ③. The hypothalamic–neurohypophyseal system is neurally connected to the neurohypophysis ④ ⑤
Regarding autonomic regulation, the electrical stimulation of almost all hypothalamic regions produces complex ANS responses (cardiovascular, respiratory, digestive, piloerection, etc.). These results are the basis for the hypothesis that the complex motor programs of the autonomic responses are contained in the hypothalamus, which are conveyed through the sympathetic and parasympathetic centers of the brainstem and spinal cord [3].
Let us take the cardiovascular function as an example. We discussed in Chap. 4 the cardiovascular servocontrol system (BP, cardiac output, vasomotor) that resides in the brainstem and acts through the autonomic segmental reactions in the spinal cord. The efferent components of this servocontrol system are the vagus nerve and sympathetic descending pathways to the intermediolateral columns of the spinal cord. The afferences come from baro- and chemoreceptors, and atrial and ventricular cardiac mechanoreceptors [4]. This level of regulation of the brainstem, self-sufficient for exclusively vascular reflexes, depends on complex stereotyped responses involving several systems under hypothalamic control. This control is exerted both by neural connections between the hypothalamus and the brainstem centers (in particular, the nucleus of the solitary tract, NTS) and by descending hypothalamic projections of the dorsal longitudinal fasciculus type [5]. The cardiovascular system is under hypothalamic control in all responses involving greater complexity than simple brainstem servomechanisms (e.g., cardiovascular changes during thermoregulation, food intake, defensive behavior) [6, 7].
The involvement of the hypothalamus in the regulation of various behaviors is revealed by the variety of responses triggered by the electrical stimulation of hypothalamic areas. Hypothalamic behaviors comprise the coordinated manifestation of neurovegetative, neuroendocrine, somatic, and motivational mechanisms. The main behaviors coordinated by the hypothalamus are: (a) defense behavior; (b) nutritive or appetitive behavior; (c) thermoregulatory behavior; (d) sexual and maternal behavior. The hypothalamus is also the site of integration of environmental and endogenous signals that determine the different biological rhythms (Chap. 2) [1].
24-h Rhythms in Neuroendocrine Function
The reactive and constitutive aspects of homeostasis (Chap. 1) are clearly manifested by studies on hormonal secretion. A variety of stimuli trigger the reactive (or facultative) secretion of a hormone, whereas periodic changes are the manifestation of the constitutive secretion (predictive homeostasis) linked to biological rhythms [8].
A broad spectrum of periodic processes characterizes the endocrine system, including infradian rhythms, whose length ranges from months to years (like the menstrual cycle or seasonal rhythms in reproduction), circadian 24-h rhythms, and secretions that occur with intervals of hours (ultradian rhythms). In many cases, ultrafast or high-frequency variations, with periods of 5–15 min, are superimposed onto the hourly variations, caused by pulsatile variations or episodic secretions of pituitary hormones.
The 24-h temporal pattern of adenohypophyseal hormone release is controlled by the circadian system (C process) and the sleep homeostat (S process) as discussed in Chap. 2 (Fig. 5.4). The daily rhythmicity of the pituitary hormones occurs mainly by the circadian modulation of the amplitude of the secretory pulses, whereas the sleep/wake homeostat exerts its influence mainly on the frequency of pulse secretion.


Fig. 5.4
The 24-h temporal pattern of adenohypophyseal hormone release is controlled by the circadian system (C process) and the sleep homeostat (S process). The daily rhythmicity of the pituitary hormones occurs mainly by the circadian modulation of the amplitude of the secretory pulses, whereas the sleep/wake homeostat exerts its influence mainly on the frequency of pulse secretion. KNDy neurons expressing kisspeptin, neurokinin B, and dynorphin. The figure was prepared in part using image vectors from Servier Medical Art (www.servier.com), licensed under the Creative Commons Attribution 3.0 Unported License (http://creativecommons.org/license/by/3.0/)
To discern the relative roles of the circadian pacemaker and the effects of sleep on daily hormonal variation, strategies are based on the notion that the circadian rhythmicity needs several days to adapt to a sudden change in the sleep/wake cycle. Studies conducted in individuals studied under normal sleep–wake cycles cannot establish whether the rhythm is circadian or is driven by external factors such as sleep–wake. A “gold standard” to disclose between the two is the constant routine protocol. Participants remain awake in bed for up to 50 h in a semirecumbent posture under dim light conditions and are fed hourly isocaloric meals.
A less precise but more practicable experimental design to discern the type of control of the constitutive secretion of a hormone is blood sampling (every 20 min) during normal sleep (day 1), night deprivation (day 2), and recovery of lost sleep from 11 am on at day 3 (Fig. 5.5) [9, 10]. By means of this strategy, it was verified that cortisol shows its maximum level at the expected time (the last part of the night and early morning) regardless of whether sleep was allowed or not (Fig. 5.5, upper panel), whereas growth hormone (GH) is secreted whenever N3 NREM sleep is detected, i.e., in the first part of the night of day 1 or during the recovery nap on day 2 (Fig. 5.5, lower panel).


Fig. 5.5
Schematic representation of a daily hormonal rhythm controlled by the circadian clock or C process (cortisol) or by the S process (growth hormone, GH). The subject was deprived of sleep for one night and could recover the lost sleep from 11 am the next day. Cortisol is secreted in the phase established by the circadian clock preceding wakefulness, in the presence or absence of sleep. GH is secreted whenever N3 sleep occurs. Data from Van Cauter and Refetoff and Copinschi et al. [9, 10]. Ultradian variations in hormone secretion and values <5 μg/dL in both hormones are not represented
For hormones controlled by the hypothalamic-pituitary axis, the pulsatile variation of their plasma levels is caused by intermittent discharges of an ultradian pulse generator (Fig. 5.4). Gonadotropin pulses were the result of gonadotropin-releasing hormone (GnRH) pulses originating from synchronous discharges of GnRH-producing neurons, controlled by a pacemaker located in the anteroventral periventricular nucleus (AVPV) and the arcuate nucleus (ARC; Fig. 5.6) [11]. In primates with hypothalamic lesions, normal levels of gonadotropins are only restored by pulsatile, but not continuous, administration of GnRH. Rather, the continuous administration of GnRH inhibits gonadotropin release. These findings have been applied to the treatment of a wide variety of disorders of the pituitary–gonadal axis, using the pulsating administration of GnRH to correct deficient production of endogenous GnRH, and the continuous administration of GnRH analogs to inhibit pituitary gonadotropin synthesis [12].


Fig. 5.6
Hypothalamic nuclei involved in the regulation of gonadotropin release . The gonadotropin-releasing hormone (GnRH) neurons of the MPOA project to the ME. Modulatory inputs upstream of the GnRH neurons include neurons that express kisspeptin (Kiss1; stimulatory of GnRH release), neurokinin B (stimulatory of GnRH release), and dynorphin (inhibitory of GnRH release; KNDy neurons). They are in the anteroventral periventricular nucleus (AVPV) and ARC. A major inhibitory signal derives from gonadotropin-inhibiting hormone (GnIH) releasing neurons of the dorsomedial hypothalamus (DMH). The SCN influences multiple sites involved in the control of gonadotropin release via monosynaptic projections from the SCN shell to the positive (KNDy and GnRH) and the negative signal of GnRH release (GnIH). The figure was prepared in part using image vectors from Servier Medical Art (www.servier.com), licensed under the Creative Commons Attribution 3.0 Unported License (http://creativecommons.org/license/by/3.0/)
The concept of the hypothalamic GnRH pulse generator emerged in the 1980s, but remained undefined for more than 20 years. The discovery of a hypogonadotropic hypogonadism associated with loss of function of GPR54, the receptor for a 54-amino acid peptide called kisspeptin (Kiss1) , which is a very potent GnRH secretagogue, changed the field dramatically. Kiss1 is critical for the onset of puberty, the regulation of sex steroid-mediated feedback, and the control of adult fertility. Further studies indicated that other two peptides, neurokinin B (stimulatory of GnRH release) and dynorphin (inhibitory of GnRH release) are co-expressed in a subset of neurons in the AVPV and ARC (Fig. 5.6). The acronym, KNDy , was coined to describe these neurons. Current knowledge holds that the hypothalamic timing mechanism is initiated in the KNDy neuronal network of the nucleus by the reciprocating interplay of stimulatory kisspeptin/neurokinin B signals and inhibitory dynorphin one (Fig. 5.6) [13]. Kisspeptin neuron activity oscillates on a circadian basis, these neurons expressing clock genes that regulate their rhythmic activities [14].
The daily rhythmicity of the pituitary hormones occurs mainly by the circadian modulation of the secretory pulse amplitude, whereas the sleep/wake homeostat exerts its influence mainly on the frequency of secretion of these pulses (Fig. 5.4). As stated, sleep dependence on rhythm is indicated because the effects of sleep occur independently of the time of day when the waking to sleep transition occurs. The persistence of rhythms in the absence of periods of sleep revealed the dependence of the circadian pacemaker on the generation of these hormonal rhythms.
Constitutive GH (Fig. 5.5) and prolactin (PRL; Fig. 5.7) secretion is controlled by the sleep/wake homeostat. In normal adult subjects, the daily profiles of GH in plasma are characterized by stable low levels interrupted abruptly by peaks of secretion [10]. Thus, GH release is pulsatile throughout the day, although in adults the most frequent secretory pulses (approximately 75% of pulses) occur in the early hours of sleep associated with slow-wave sleep (N3), with very low secretory activity during REM sleep.


Fig. 5.7
Schematic representation of the daily rhythm of prolactin (PRL; upper panel) controlled by the S process, and that of thyroid-stimulating hormone (TSH), under the double control of C and S processes (lower panel). The volunteer was deprived of sleep for one night and could recover the lost sleep from 11 am the next day. Ultradian variations in hormone secretion or values <100% of mean plasma PRL or <2 μU of TSH/mL plasma were not represented. Data from Copinschi et al. [10]
The episodic secretion of GH depends on the specific rhythms of growth hormone-releasing hormone (GHRH) and on the hypothalamic GH inhibitory hormone, somatostatin , whose releases are 180 degrees out of phase. Variations in the secretion of somatostatin explain different sensitivities to GHRH during sleep [15]. Thus, stage N3 sleep is associated with low levels of hypothalamic somatostatin and REM sleep with high levels. Somatostatin secretion fixes the time (frequency and duration) of the release of GH, whereas the secretion of GHRH determines its magnitude. Humans are the only species that shows a close relationship between GH secretion and sleep. It has been suggested that the association between sleep and GH secretion in humans is the result of the consolidation of the sleep–wake cycle [16, 17].
The total amount of GH secreted daily is closely related to the individual’s age. In the prepubertal phase, GH pulses occur predominantly between 22:00 and 04:00 h, whereas at puberty the GH pulses are larger in number and amplitude, distributing throughout the 24-h period. Prepubertal children have daily mean values of GH concentration like those post-puberty and in adult life, whereas at the end of puberty, the total amount of GH secreted daily reaches its maximum value. As age increases, nocturnal GH pulses decrease in frequency and amplitude, with no appreciable changes in plasma GH concentration during the day [10].
In normal young adult males, most of the daily GH secretion (>70%) occurs shortly after sleep onset during NREM N3 sleep. In young women, there are also pulses during the day that are more frequent and of greater amplitude than the men, related to the estrogens. From the age of 50–60 years, there is no constitutive release of GH, which is responsible for the loss of lean muscle mass (about 2 kg per decade); this coincides with the disappearance of N3 sleep. The major primary alteration that accounts for GH hyposecretion in old age is the increased secretion of hypothalamic somatostatin. There is also a decrease in the effectiveness of GHRH at releasing GH. Women generally secrete more GH than men of the same age and in women the effect of aging on GH secretion correlates with the decrease in circulating estradiol concentration [17].
Under normal conditions, the daily profile of PRL plasma levels follows a pattern with minimal morning concentrations, a gradual increase in the afternoon, and a higher nocturnal elevation that begins after the onset of sleep and culminates after around half of it [18]. This is outlined in Fig. 5.7, top panel. In addition, over 24 h, there are episodic pulses of PRL that can range from 7 to 24 throughout the day. Modulation of the amplitude of the secretory pulses gives rise to the daily pattern of hormonal secretion.
The constitutive secretion of PRL is strongly related to sleep homeostasis, as daytime sleep or naps are associated with an increase in the release of the hormone. Similarly, studies after reversal of the sleep–wake cycle have shown that sleep onset is associated with an increase in PRL secretion, whereas sleep deprivation prevents a nocturnal increase in PRL . However, in changes in the sleep–wake cycle and in sleep deprivation, it has been shown that the temporary organization of PRL release also presents some inherent circadian rhythmicity shown by a slight hormonal elevation corresponding to the moment of omitted sleep. PRL secretion plays a potential role in circadian REM sleep and N3 sleep control, which increases in patients with hyperprolactinemia and in lactating women [17].
The circadian clock controls the constitutive secretion of cortisol and melatonin . The periodicity of 24 h in the secretion of the pituitary–adrenal axis is considered a paradigm of circadian rhythmicity in humans (Fig. 5.4, upper panel). It begins at the age of 6 months and persists in adults until old age. The daily profiles of plasma cortisol are parallel and show a 2–3 h delay to those of adrenocorticotropic hormone (ACTH) . This pattern shows a maximum at the beginning of the morning (04:00–08:00 h), a decrease throughout the day, followed by a low concentration during the night period (giving rise to a quiescent period from 24:00 at 02:00 h) and an abrupt rise at the end of the sleep period [19].
During the 24 h, about 15 pulses of cortisol and ACTH occur, which indicates that the daily variation of cortisol levels reflects a modulation of the amplitude of the pulses rather than the frequency. That is, the 24-h rhythm of cortisol is mainly dependent on the circadian pattern of ACTH release , which is amplified by a daily variation in the adrenal response to it. This depends on the autonomic innervation of the adrenal, a case like that described for thyroid innervation in Chap. 4. In turn, the rate of ACTH release is the result of periodic changes in the release of corticotropin-releasing hormone (CRH) [19].
The rhythm of secretion of the hypophyseal–adrenal axis persists in elderly subjects, although in general, the elevation in cortisol levels occurs earlier, with reduced latency to REM sleep. In addition, an increase in free plasma cortisol levels has been observed in older people, attributed to a decrease in the concentration of transport proteins and/or a decrease in the binding capacity of these proteins [18].
The circadian pacemaker directly controls the daily rhythm of cortisol, as demonstrated by the rapid phase shift observed after exposure to bright light at certain circadian moments. This rhythmicity of cortisol , which is of an endogenous nature, persists during sleep deprivation, although a reduction of 10–20% of the amplitude is observed. Nighttime sleep exerts an inhibitory effect on the secretion of cortisol, superimposed on its endogenous circadian rhythmicity [20].
In old age, free cortisol levels increase by 20–50% compared with those in young people, and typically, the minimum (nadir) of the rhythm of cortisol in an individual over 70 years old is higher than that of a young adult. There is also a phase advancement of the rhythm of cortisol with age [18]. Studies in animals and in human clinics have indicated important neurodegenerative effects of cortisol, especially at hippocampal levels, which are more pronounced in the nadir of the rhythm than in its crest. We will discuss them further in this Chapter. Therefore, even modest elevations of evening cortisol in the elderly facilitate the development of disturbances associated with excess glucocorticoids, such as memory deficit and insulin resistance associated with old age [18].
The plasma concentration of melatonin has peak nocturnal values ranging from 0.4 to 0.8 pmol/mL and a minimum during the day (0.02 pmol/mL) in humans. In saliva , its values are 0.6–0.8 pmol/mL at the time of the nocturnal maximum (Fig. 5.8). In man, peak melatonin secretion is not related to the sleep phase. In the same way as cortisol, the daily rhythm of melatonin secretion remains in conditions of sleep deprivation (Fig. 5.8). Owing to its marked intraindividual reproducibility, the rise in melatonin in the afternoon in dim light (dim light melatonin onset, DLMO) is the most accurate marker of τ, the circadian rhythm period (Fig. 5.8) [21].


Fig. 5.8
Saliva melaton in levels in a volunteer subjected to a night of sleep deprivation. Saliva samples were taken every 20 min. Melatonin was assayed by radioimmunoassay. DMLO dim light melatonin onset. Values of melatonin <0.01 pmol/mL of saliva are not depicted. Cardinali et al., unpublished data
The hypothalamic–pituitary–thyroid axis presents a complicated temporal structure with rhythmic variations of multiple frequencies at all levels of the system, from the hypothalamic neurons to the cells of the peripheral effector tissues. The range of frequencies consists from rapid neural discharges to circadian and circannual rhythms, superposing on these rhythmic variations according to age. Rhythmic and nonrhythmic variations interact, modulate, and are modulated by variations of other neuroendocrine, metabolic, and immune functions. In normal subjects, thyroid-stimulating hormone (TSH) is secreted from the pituitary in a pulsatile form, demonstrating that the pituitary secretion of TSH responds better to an intermittent continuous stimulation of thyrotropin-releasing hormone (TRH) . The average frequency is nine pulses in 24 h, increasing in amplitude and frequency at night, which leads to a nocturnal rise in hormone levels [22].
Under normal conditions, TSH levels are low throughout the day, experiencing a sudden increase at 20:00 h, reaching the maximum levels at night, this maximum being circadian pacemaker-controlled (Fig. 5.7, lower panel) [23]. High hormone levels remain throughout the night, corresponding to an increase of approximately 175% of daytime levels, to decrease rapidly in the morning, reaching a nadir in the early morning hours.
The existence of circadian rhythms of thyroid hormones is debatable. A 12-h hemicircadian rhythm with morning and evening minima may occur [24]. It has been suggested that these variations of low amplitude might be related to daily variations in plasma proteins dependent on posture. As we discussed in Chap. 4, autonomic innervation plays a major role in the modulation of the thyroid response to TSH and may be responsible for this dissociation between TSH and thyroid hormone rhythms.
To investigate the role played by sleep in the daily pattern of TSH, sleep deprivation or sleep–wake cycle inversion indicate, contrary to prediction, an inhibitory influence of sleep [23]. The inhibitory influence of sleep on TSH secretion is evident during sleep deprivation, more than doubling the increase in nocturnal values (Fig. 5.7).
The daily variation of PTH is characterized by an increase in the mean nocturnal levels, with a temporal interrelation between blood concentrations of PTH and calcium. Plasma PTH levels are normally high during the night and early morning and lowest at approximately 10:00 h [25]. Concerning 1,25-dihydroxycholecalciferol, results are conflicting on the existence of circadian variations. Small daily fluctuations in calcitonin concentration have been reported in humans with increased calcitonin level during the afternoon. Food intake influences serum calcitonin level in healthy young subjects.
Bone resorption is closely linked to sleep quality. Bone cells exhibit 24-h cycles with nocturnal expression of genes that regulate osteoblast function, bone mineralization and ossification, and bone resorption markers. Interruption in melatonin-mediated signaling is responsible for the increased risk of hip and wrist fracture and low bone density in shift workers (Fig. 5.9) [21].


Fig. 5.9
Relationship between melatonin secretion and bone resorption in a 24-h cycle. As the image shows, both bone resorption (dotted line) and melatonin (solid line) show a daily rhythm, with peaks occurring during dark hours. Suppression of nocturnal melatonin levels, either by exposure to light at night (LAN) or in aging, increases bone resorption. The restoration of nocturnal melatonin maximum protects the bone loss
By means of studies in volunteers in the sleep laboratory to which several neuropeptides were administered in pulsatile form, the link between neuropeptides and the different stages of sleep determined by polysomnography (PSG) could be verified [26]. During the first half of the night and coincident with slow-wave sleep, the mechanisms associated with GH secretion predominate, whereas in the second half of the night there is a coincidence in the percentage increase in REM sleep with the secretion of the hypothalamic–pituitary–adrenal axis hormones. Based on this, the homology of GH secretion with the S process and activation of the ACTH/cortisol axis with the C process has been proposed (Fig. 5.10). Neuropeptide perfusion studies indicated that: (a) GHRH promotes GH release and slow-wave sleep; (b) CRH promotes the activation of the pituitary–adrenal axis and REM sleep; (c) galanin increases slow-wave sleep in the absence of changes in GH and cortisol; (d) somatostatin inhibits GH and slow-wave sleep and promotes REM sleep; (e) NPY is an endogenous CRH antagonist and contributes to fixing the time of onset of sleep; (f) ghrelin increases GH, NREM sleep, and cortisol [26].


Fig. 5.10
Neuropeptide perfusion studies in healthy volunteers during a PSG show that GHRH perfusion promotes GH release and NREM sleep, whereas infusion of corticotropin-releasing hormone (CRH) promotes pituitary–adrenal axis activation and REM sleep. A reciprocal interaction of GHRH and CRH as a key to sleep regulation was proposed. GHRH predominates during the first half of the night, resulting in slow sleep, GH secretion, and minimal secretion of adrenocorticotropic hormone (ACTH) and cortisol, whereas the second half of the night is dominated by CRH (ACTH and cortisol secretion). These periods are superimposed on the results of an experiment in which a healthy volunteer was deprived of sleep for one night and could recover the lost sleep from 11 am the next day. Modified with permission from Cardinali [1]
It should be noted that changes in EEG of sleep following administration of GHRH and CRH are not due to GH or cortisol secretion, as slow sleep decreases after GH administration, whereas slow-wave sleep and GH increase after injecting cortisol. Therefore, the results are best explained by the inhibitory feedback on GHRH and CRH respectively.
These studies have been of interest in linking normal aging with depression [26]. In both situations, there is deterioration of slow-wave sleep, shortened REM sleep and increases in REM sleep, in addition to changes in the continuity of sleep (prolonged sleep latency, frequent nocturnal awakenings, early morning awakening). Endocrine changes are similar in both situations: there is an elevation of ACTH and cortisol and suppression of GH. This is because the GHRH/CRH ratio changes in favor of CRH during an episode of depression because of CRH hypersecretion , whereas in aging the GHRH/CRH ratio changes in favor of CRH because of the reduction of GHRH activity [26].
The rhythmic aspects of the activity of the hypothalamic–pituitary–gonadal axis, the neurohypophyseal system, and the secretion of hormones associated with energy homeostasis and blood volume control are discussed below with their specific behaviors.
Defense Behavior as a Paradigm of Reactive Homeostasis
Nothing in physiology illustrates the concept of reactive homeostasis better than defense behavior. Hans Selye named it “general adaptation syndrome ” to define the set of changes arising as the body’s response to a wide variety of noxious stimuli (stressors) [27]. Stressors are those stimuli whose perception by the nervous system does not match the neural representation of past experiences, and to which a change in coping strategy (e.g., a particular behavior) is not successful (Fig. 5.11).


Fig. 5.11
Behavioral and biological aspects of the defense behavior. The sequence of phenomena following exposure to a novel situation is described. Strategies are of two types, high or low energy level. Their objective is adaptation. If adaptation does not occur, the stress reaction appears, with stimulation of the pituitary–adrenal axis
In general, the stimuli stressors can be classified into four groups: (a) physical/chemical stressors (heat, cold, intense radiation, noise, vibration, toxic substances, etc.); (b) psychological stressors (emotional and behavioral changes, such as anxiety, fear, frustration); (c) social stressors (hostile environment, disruption of relations); (d) those that alter ANS homeostasis (exercise, orthostatic, body tilt, hypoglycemia, bleeding, etc.).
An animal that is faced with a threatening situation must decide, among different strategies, what is apparently the best choice for the conservation of its life and, in a broad sense, of its species. For example, when sympathetic activation occurs, cardiac output and systemic blood flow increase and, although vasodilation occurs to increase the blood supply to active skeletal muscles for posture and intended movements, vasoconstriction in the skin can reduce blood loss in a region that is susceptible to damage [28]. A decreased time for blood clotting avoids a large hemorrhage, and higher sudoresis makes the skin moistened and the animal difficult to catch. At the same time, there is enhanced breathing movements and airflow volume for gaseous exchanges and pH regulation. Liver glycogenolysis is stimulated, along with the mobilization of fatty acids from adipose tissue [29]. Muscular strength and muscle glycolysis are also improved and, for additional energy production, a higher oxygen–hemoglobin dissociation and oxygen delivery to activated cells also occur. Wave frequencies in the EEG increase, which reflects diffuse neuronal activation, and mydriasis serves to provide more visual information and to decide the best behavioral strategy to execute, such as skipping out of a dangerous place. By the action of α-adrenoceptor receptors, the secretion of insulin is inhibited (it would be useless to restore energy reserves at this moment), whereas β-adrenoceptors stimulate glucagon secretion. Cortisol, in addition to other stress-related hormones, can indirectly affect the consolidation of memories. Cells of the immunological system, lymphoid tissues, and cytokines are also involved in this integrated response (Fig. 5.12).


Fig. 5.12
Via ACTH release and activation of adrenal sympathetic nerves, the defense behavior is developed. Modified with permission from Cardinali [1]
Thus, coping with stressors includes two strategies (Figs. 5.11 and 5.13). An active coping strategy (fight-or-flight) is evoked if the stress is predictable, controllable, or escapable. A passive coping strategy (immobility or decreased responsiveness to the environment) is evoked if the stress is inescapable [1].


Fig. 5.13
The different neuroendocrine profiles of defense behavior
The active strategy is associated with sympathoexcitation (hypertension, tachycardia, thermogenesis), whereas the passive strategy is associated with sympathoinhibition and/or parasympathetic activation (hypotension, bradycardia). The passive strategy also helps to facilitate recovery and healing.
The active strategy is called the “fight-or-flight” response from a behavioral point of view or the “defense response” from an autonomic point of view. The passive strategy is sometimes called “paradoxical fear ” or “playing dead.” Parts of the neural substrates that mediate active versus passive emotional coping have been identified within the brainstem [30, 31].
If strategies to new situations (high- or low-energy consumption) are not successful, the stress reaction is triggered (Figs. 5.10 and 5.12). The responses correspond to different functional configurations of the limbic system (amygdala dominance in the fight situation; predominance of septo-hippocampal components in defeat; Fig. 5.13).
During stress, the physiological processes that do not pose a short-term benefit are inhibited, such as inflammation, digestion, reproduction, and growth. When the intensity or duration of stressors exceed certain limits, pathological changes such as hypertension, gastric ulcers or neurological abnormalities ensue.
The defense capability or adaptation of an organism to stress depends on the magnitude and duration of the stressor, and on the sensitivity to the stressor. There are differences in the ways in which individuals face situations of stress. These differences are related to genetics, environmental influences, previous experience in such situations, training, social support, and physical and mental health. An important difference is the programming of ANS connections during early extrauterine life (Fig. 5.14) [32]. The conditions of reaction to stress have clear individual nuances, arising from fixed initial experiences in a learning phase, during the early stages of life. This explains why the same stressful situation, which is not harmful to many individuals, leads some to myocardial infarction, others to peptic ulcer or ulcerative colitis, and others to hyperthyroidism. That is, the responses of the ANS depend on the previous history and individual experience.


Fig. 5.14
There are differences in the ways in which individuals face stressful situations. These differences are related to genetics, environmental influences, previous experience in such situations, training, social support, and physical and mental health. An important difference is the programming of ANS connections during early extrauterine life. Modified with permission from Cardinali [1]
Stress responses involve a complex neurobehavioral cascade , which is elicited when the organism is confronted with a potentially harmful stimulus. As this stress cascade consists of a range of neural and endocrine pathways, stress can be conceptualized as a communication process on the descending branch of the brain–body axis. Therefore, interoception and stress are associated via the bi-directional transmission of information on the brain–body axis. The excessive and/or enduring activation (e.g., by acute or chronic stress) of neural circuits, which are responsible for successful communication on the brain–body axis, induces malfunction and dysregulation of these information processes. Therefore, interoceptive signal processing may be altered, resulting in physical symptoms contributing to the development and/or maintenance of body-related mental disorders, which are associated with stress. A positive feedback model involving stress (early life or chronic stress, and major adverse events), the dysregulation of physiological stress axes, altered perception of bodily sensations, and the generation of physical symptoms, which may in turn facilitate stress, have been proposed [33, 34] (Fig. 5.14).
In the event of a challenge to homeostasis (stress), recovery may occur with disappearance of the stressor. For example, in a high salt diet, homeostatic mechanisms promote the elimination of excess sodium to restore equilibrium. However, even in situations when the stressor is not eliminated, it is possible to restore and maintain homeostasis (Fig. 5.15). To achieve this, animals must have to preserve the stability of their variables through changes of state related to challenging circumstances. In this sense, the concept of “allostasis ” (allo, meaning different, and stasis, meaning constancy, i.e., “achieving stability through change”) was introduced to take into account the regulatory systems that develop variable set points of control, showing individual differences in expression according to the capacity of the animal to cope with new situations [30]. This is associated with anticipatory behavioral and physiological responses, and is vulnerable to physiological overload and the breakdown of regulatory capacities.


Fig. 5.15
In the event of a challenge to homeostasis (stress), recovery may occur with disappearance of the stressor (left). However, even in situations when the stressor is not eliminated, it is possible to restore and maintain homeostasis (right). The concept of “allostasis ” was introduced to consider the regulatory systems that develop variable set points of control, showing individual differences in expression according to the capacity of the animal to cope with new situations. The figure was prepared in part using image vectors from Servier Medical Art (www.servier.com), licensed under the Creative Commons Attribution 3.0 Unported License (http://creativecommons.org/license/by/3.0/)
The allostatic mechanisms can maintain homeostasis in the presence of a stressor (Figs. 5.15 and 5.16). As important as the mobilization of functional and behavioral processes to maintain the homeostatic balance in response to the stressor, is the appropriate demobilization of these processes when terminating the stressor stimulus. Thus, both the mobilization and the inadequate demobilization of allostatic mechanisms (Fig. 5.16) can cause adaptation diseases, such as hypertension, obesity, diabetes, stroke, autoimmune diseases, inflammatory disorders, and gastric ulceration [30].


Fig. 5.16
Allostatic load. The upper panel illustrates the normal allostatic response, which begins with a stressor-induced response, maintained for a suitable period, and then reversed. The remaining panels illustrate four conditions that give rise to allostatic load: (1) repetitive appearance of multiple stressors (e.g., repeated elevation of BP leads to arteriosclerosis); (2) lack of adaptation (e.g., those individuals who have to speak in public and do not reduce cortisolemia with repetition); (3) Prolonged responses due to a delayed reversal (e.g., catecholamine and cortisol secretion due to stress returns to basal levels more slowly in the elderly), (4) an inadequate, low response resulting in the compensatory hyperactivity of other mediators (e.g., autoimmune disorders with inadequate secretion of corticosteroids and a high concentration of cytokines, which are usually counter-regulated by corticosteroids). Modified with permission from Cardinali [1]
The coping responses during stress can be defined as cognitive and behavioral responses to managing the stress. The primary objectives of the success of coping responses are: to eliminate or mitigate the harmful environmental conditions and to enhance the prospect of recovery; to tolerate and adjust the body to negative events; to maintain a positive self-image; to maintain emotional balance; and to preserve social relations [35].
Sleep implies a recovery process from previous wakefulness. Not only the duration of the waking period affects sleep architecture and sleep EEG, the quality of wakefulness is also highly important. Studies in rats have shown that social defeat stress, in which experimental animals are attacked and defeated by a dominant conspecific, is followed by an acute increase in NREM sleep on EEG and the suppression of REM sleep. This occurred in both winners and losers, indicating that in rodents a social conflict with an unpredictable outcome has quantitatively and qualitatively similar acute effects on subsequent sleep, regardless of the results of the conflict [36].
There is evidence that acute or chronic exposure to a stressor can start or cause recurrence of psychiatric disorders such as depression, bipolar disorder, post-traumatic stress disorder, anxiety, and schizophrenia. In situations of melancholic depression, anorexia nervosa, panicky anxiety, obsessive–compulsive disorder, chronic active alcoholism, excessive exercise, abstinence from alcohol and narcotics, malnutrition, sexual abuse, the chronic activation of the hypothalamic–pituitary–adrenal axis occur. On the other hand, in situations such as seasonal or atypical depression, the postpartum period, the period after smoking cessation, fibromyalgia syndrome or chronic fatigue, CRH secretion is diminished and symptoms occur such as increased appetite and weight gain, somnolence, and fatigue [37, 38].
The parvocellular neurons of the hypothalamic PVN that synthesize and release CRH and/or AVP are the final common pathway for the regulation of ACTH secretion (Fig. 5.17) [30]. The relative proportion of active CRH/AVP neurons increases significantly in stress [39]. Among other brain areas, these neurons project the median eminence, to noradrenergic neurons of the brainstem, and to the ARC. In the latter, they activate pro-opiomelanocortin -containing neurons, which, in turn, reciprocally innervate CRH/AVP PVN neurons. Dendritic release of neuropeptides has been described as a novel interpopulation signaling modality in the PVN [40].


Fig. 5.17
The CRH neurons of the paraventricular nucleus of the hypothalamus command the different manifestations of defense behavior. Modified with permission from Cardinali [1]
A high density of neuronal cell bodies containing CRH is also found in the central amygdaloid nucleus and the bed nucleus of the stria terminalis. CRH and its receptors are found in extra-hypothalamic structures such as the limbic system, basal forebrain, PBN, and paragigantocellular nuclei of the medulla oblongata, LC, and other groups of noradrenergic neurons of the pons and medulla (sympathetic noradrenergic system) and spinal cord [39].
There are reciprocal neural connections between CRH neurons in the PVN and noradrenergic neurons in the brainstem. Cholinergic, serotonergic, and glutamatergic neurons have stimulatory action in those circuits. The brainstem catecholaminergic pathways to the PVN CRH neurons can be activated in bleeding, hypotension, respiratory distress, and immune challenges. The LC is one of the regions of the brain that is most responsive to stress, especially to hemorrhage. It also exerts its effects on the hypothalamic–pituitary–adrenal axis through central limbic structures and it innervates the PVN directly.
Glucocorticoids have both direct and indirect inhibitory actions on PVN neurons. The hippocampus , which shows large numbers of binding sites for glucocorticoids and mineralocorticoids, is one of the structures that inhibit PVN activity [19]. Hippocampal damage increases mRNA expression for CRH and AVP in the PVN and hippocampal stimulation decreases the activity of the hypothalamic–pituitary–adrenal axis in rats and humans. The lateral septal area, the mPOA , and prefrontal cortex are also structures that have inhibitory actions on the PVN.
The role of the medial prefrontal cortex as a coordinator of behavioral and physiological stress responses across multiple temporal and contextual domains has been proposed [41]. Glucocorticoids act as one of the primary messengers in the reallocation of energetic resources, having profound effects locally within the medial prefrontal cortex, and shaping how the brain region acts within a network of brain structures to modulate responses to stress.
Several neurotransmitters are released in the brain during stress, but CRH is the main coordinator of the psychological and behavioral changes found (Fig. 5.17) [31]. Experimental studies have shown that exogenous administration of CRH stimulates the release of ACTH by the pituitary, can change the EEG, and induces psychological and behavioral changes such as those observed in stress, for example, reduced feeding behavior. Hormones of the pituitary–adrenal axis do not block behavioral effects, but they can be reversed by the neutralization of CRH, indicating that CRH can modulate behaviors independently of the pituitary–adrenal axis (Fig. 5.18).


Fig. 5.18
Partly for genetic and epigenetic reasons, a vulnerable phenotype of CRH hyper-responsiveness to stress leads to emotional disorders. The use of pharmacological agents or psychotherapy counteracts the consequences of this phenotype. HPA pituitary-adrenal axis. Modified with permission from Cardinali [1]
Therefore CRH produces activation of ANS via direct central action, rather than through the activity of the hypothalamic–pituitary–adrenal axis. CRH is part of a family of peptides that includes urocortin and urotensin. These peptides act via two receptor types: CRH-R1 and CRH-R2. The CRH-R1 receptors are most abundant in the brain and in peripheral tissues and have a higher affinity for CRH and urocortin. The CRH-R2 receptors are less abundant in the nervous system, are more significant in peripheral tissues and exhibit higher affinity for urocortin, urocortin II, and urocortin III than for CRH [31].
The predominant receptor in the activation of the hypothalamic–pituitary–adrenal axis is CRH-R1, whereas CRH-R2 plays a prominent role in the energy expenditure processes involved in homeostatic responses. Animals genetically deficient in CRH-R1 show a failed stress response, whereas those deficient in CRH-R2 are hypersensitive to stress and exhibit increased anxiety. It is possible that the acute stress response phase is linked to rCRH-1, whereas the final recovery stage includes rCRH-2 effects. Selective antagonists of rCRH-1 inhibit the anxiogenic action of CRH and have been proposed to be a new type of anxiolytics. Hypersecretion of CRH is as a phenotypic element of emotional disease vulnerability (Fig. 5.18). Significance has also been given to the early effects of glucocorticoid hypersecretion, with lasting changes in these systems in the life of the individual [31].
The hierarchical relationship between the hypothalamus and the limbic system that controls it as the last level of autonomic motor hierarchy is clear when the defense behavior is tested. An animal bearing a hypothalamic deafferentation that disconnects the limbic system responds to the appearance in the visual field of any object with the reaction of “false rage.” This indicates that the hypothalamus contains the “program” of the defense reaction, which acquires emotional meaning and purpose under the control of the limbic system. Maintaining high levels of cortisol in stress leads to verifiable chronic damage of hippocampal neurons with significant cognitive deficits (Fig. 5.19) [30, 42].


Fig. 5.19
Glucocorticoids and hippocampal neurogenesis . Several factors are listed that decrease or increase neurogenesis. MR, GR receptors for mineralo- and glucocorticoids. Modified with permission from Cardinali [1]
Stress interferes with the hypothalamic circuitry regulators of appetite and satiety. CRH is a potent anorexic substance whereas NPY is the most potent known orexinergic substance. NPY neurons stimulate CRH neurons, constituting a feedback loop involved in the control of food intake. Simultaneously, NPY inhibits the LC, and stimulates food intake [43]. Glucocorticoids induce gluconeogenesis and insulin resistance, by inhibiting lipolytic enzymes and stimulating enzymes that facilitate the deposition of fat, thus antagonizing the actions of GH and gonadal hormones on lipolysis and muscle and bone anabolism. Chronic stress can cause increased visceral adiposity, the suppression of osteoblastic activity, and the reduction of lean mass (muscle and bone). Monkeys subjected to chronic social stress show the classical symptoms of metabolic syndrome, such as high deposition of visceral fat, higher incidence of coronary atherosclerosis, hyperglycemia, and insulin resistance. Hypertension, adrenal hypertrophy, hypersensitivity to adrenal ACTH, hypogonadism and high cholesterol and lipid levels in the blood are also found. The correlation between chronic stress and metabolic syndrome is also observed in humans [44]. The phenotype of central or visceral obesity and a decrease in lean body mass are seen in patients with Cushing’s syndrome, and in patients with melancholic depression and chronic anxiety, all situations of hypercortisolemia. Visceral adiposity establishes a vicious cycle of an increased need for insulin, hyperglycemia, and hypercholesterolemia.
Chronic stress affects therapeutic efficacy in cancer. For example, epidemiological studies revealed strong correlations between long-term survival/cancer progression and β-adrenoceptor blocker use in patients [45, 46]. The contributions of stress to immunosuppression in the tumor microenvironment and the implications of these findings for the efficacy of immunotherapies have been discussed [47]. Effects of norepinephrine (NE) on immune cells, such as those discussed in Fig. 4.25, are presumably involved.
Studies in rodent tumor models have identified adrenergic receptor expression on various cancer cells including mammary carcinogen-induced tumors, melanoma, and pituitary tumors. In humans, the Ewing sarcoma, neuroblastoma, rhabdomyosarcoma, lymphoma, melanoma, and pancreatic, lung, breast, and prostate cancer cells all displayed detectable levels of adrenoceptors. Several studies demonstrate that the activation of adrenergic receptors promotes tumor progression. For example, chronic activation of G protein-coupled receptors, such as the α1B-adrenergic receptor, can induce malignant transformation in normal cell lines, promote DNA damage, and enhance tumor formation. In addition to a role in cell survival, adrenergic receptor signaling has also been widely studied for mediating metastasis. The regulation of metastasis by adrenergic receptors occurs at multiple levels and involves not only cancer cells, but also cells in the tumor microenvironment and in the metastatic niche. [47, 48]. Sympathetic activation modulates gene expression programs that promote the metastasis of solid tumors by stimulating macrophage infiltration, inflammation, angiogenesis, epithelial–mesenchymal transition, and tumor invasion, and by inhibiting cellular immune responses and programmed cell death. Hematological cancers are modulated by ANS regulation of stem cell biology and hematopoietic differentiation programs [49].
In the author’s laboratory, two breast cancer tumor lines were used to assess the reactivity of sympathetically denervated murine skin [50]. M3 tumors had a relatively high capacity for local growth and a low capacity for metastasis, whereas MM3-LN tumors grew locally at a slower rate, but metastasize very early to the lung. After local implantation in the ear, the growth of M3 and MM3-LN tumors was significantly slowed in the previously sympathetically denervated skin territory; however, their metastatic capacity remained unaffected by SCGx [50].
Stress related to inflammatory and infectious processes can alter the reproductive function by the inhibitory action of substances released by the immune system cells on the hypothalamic–pituitary–gonadal axis [51]. CRH suppresses GnRH release, whereas glucocorticoids act on the pituitary and gonads causing inhibition of the secretion of luteinizing hormone (LH), follicle-stimulating hormone (FSH), and gonadal steroids . In stressed women, a decreased frequency of LH pulses occurs together with increased cortisol secretion, which are assigned respectively to the decreased activity of the GnRH pulse generator and increased CRH activity. The central administration of cytokines reduces plasma LH levels, probably by increasing opioids and prostaglandins, which inhibit the secretion of GnRH. Additionally, cytokines can inhibit the activity of enzymes necessary for gonadal steroidogenesis.
The interaction of immune activity with the stress system is illustrated by the fact that vaccination may not be completely effective at establishing the immune defense to immunization that occurs during stress. Antigens are stressful stimuli (noncognitive), and the antigenic challenge features a stressful situation (Fig. 5.20). Infection stressor is a stimulus that induces the production of cytokines by the immune system and glial cells. Inflammatory and infectious processes stimulate visceral vagal afferents that activate the CRH stress system via the LC [52]. The hyperactivation of the hypothalamic–pituitary–adrenal axis induces the hypersecretion of glucocorticoids and immunosuppression, which are among the main consequences of stress (Fig. 5.20). The hypothalamic–pituitary–adrenal axis produces glucocorticoids, inhibiting the immune system. This, in turn, produces cytokines that are stimulatory for CRH release. Thus, this constitutes a mechanism for feedback between the neuroendocrine and immune components in stress. The prevalence of the neuroendocrine component causes immunosuppression, whereas the prevalence of the immune component causes autoimmune disease [19, 53].


Fig. 5.20
Antigens are stressful stimuli (noncognitive), and the antigenic challenge features a stressful situation
The above-mentioned mechanisms also include the peripheral portion of the ANS. From studies in the SCG territory , it can be concluded that during the augmented NE release from peripheral sympathetic nerves innervating the hypothalamic–hypophyseal unit, the modulatory role of peripheral nerve terminals is differentially exerted on the release of hypophysiotropic hormones, inhibiting the release of all pituitary hormones, except for ACTH (Fig. 5.21) [54]. In animals with chronic SCGx, both ACTH and corticosterone rhythms were suppressed. The mechanism through which peripheral sympathetic neurons are capable of modifying the function of the median eminence remains undefined. As SCG efferences play an important role in regulating the cerebral and choroidal blood flow, the outcomes of SCGx on the median eminence may represent a particularly sensitive vasomotor effect of the peripheral noradrenergic terminals. Otherwise, they may involve a more complex interrelationship, such as a direct modulatory effect on hypophysiotropic hormone release [54].


Fig. 5.21
Diagram that summarizes the changes in the hypothalamic–pituitary unit after cervical sympathetic activation. SON supraoptic nucleus, PVN paraventricular nucleus, SCG superior cervical ganglion, MCG medial cervical ganglion, ICG inferior cervical ganglion. Reproduced with permission from Cardinali [21]
24-h Rhythms in Food Intake, Energy Storage, and Metabolism
The first observations about daily changes related to nutrition and feeding were those of Sanctorius in the seventeenth century, who conducted the first autorhythmometry study by building a large scale in which he lived for months, recording his weight and the ingested food, and collecting his feces and urine. Among other observations, he detected a daily rhythm in body weight.
The complexity of the circadian system, described in Chap. 2, is exemplified when the rhythmicity of food and nutrition is studied (Fig. 5.22). The 24-h rhythm in feeding activity is controlled by the SCN . On the one hand, feeding time has synchronizing effects on the circadian system and food restriction can entrain certain rhythms through a food-related pacemaker. Finally, food, besides being a synchronizer and a variable controlled by the clock, food is also a masking stimulus that modifies many rhythms related to digestion, absorption, and metabolism of nutrients.


Fig. 5.22
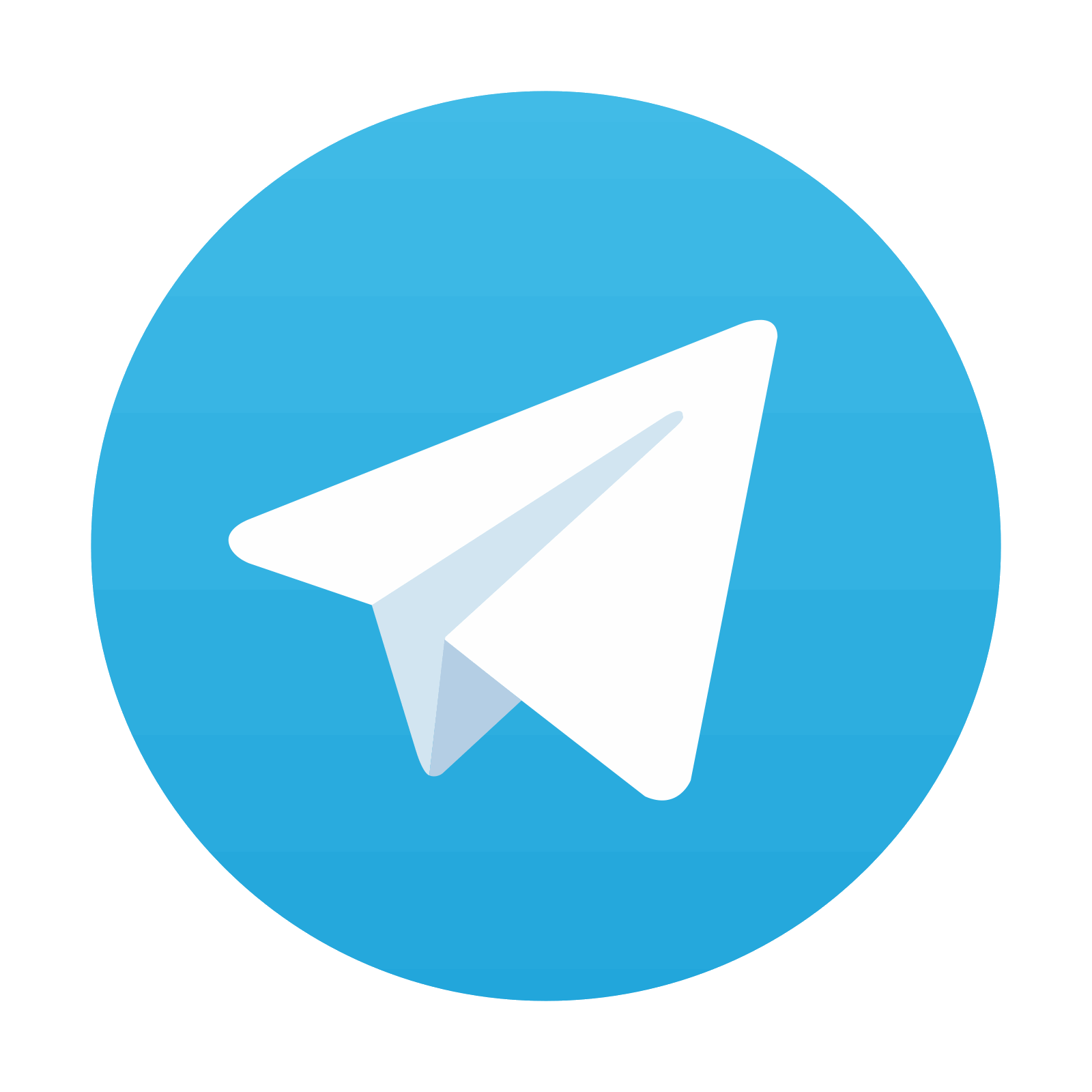
Rhythmicity of feeding and nutrition. On the one hand, the feeding time has synchronizing effects; on the other, the food restriction can synchronize certain rhythms through a food-controlled pacemaker. Food activity is in turn controlled by the SCN. In addition to being a synchronizer and a variable controlled by the clock, food is a masking agent to directly modify numerous rhythms related to the digestion, absorption, and metabolism of nutrients. The figure was prepared in part using image vectors from Servier Medical Art (www.servier.com), licensed under the Creative Commons Attribution 3.0 Unported License (http://creativecommons.org/license/by/3.0/)
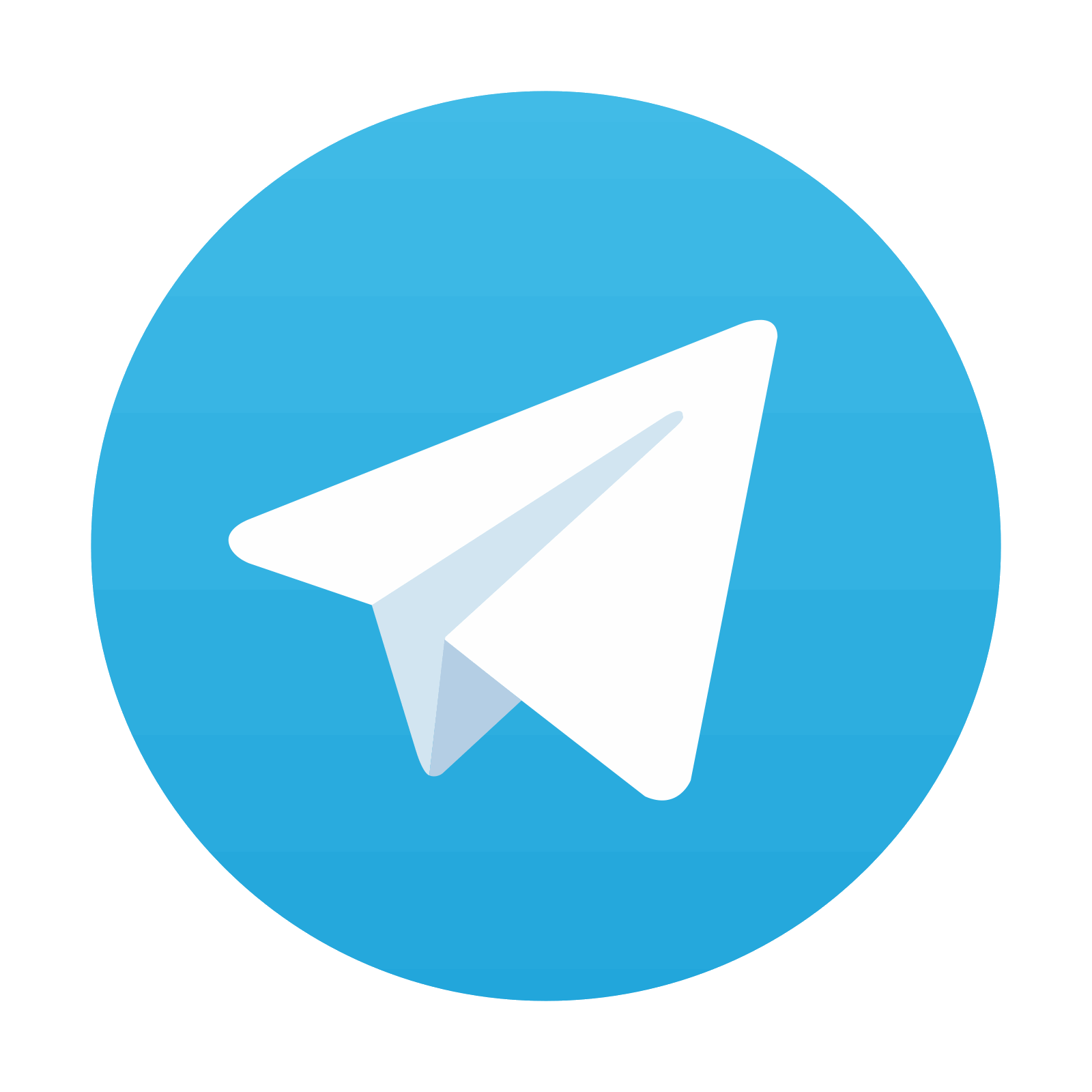
Stay updated, free articles. Join our Telegram channel
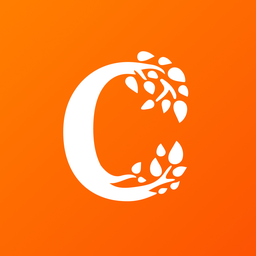
Full access? Get Clinical Tree
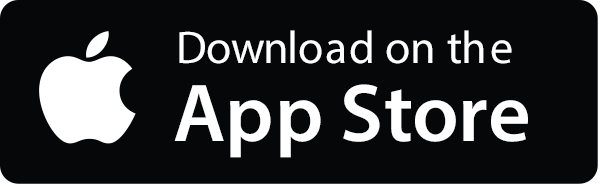
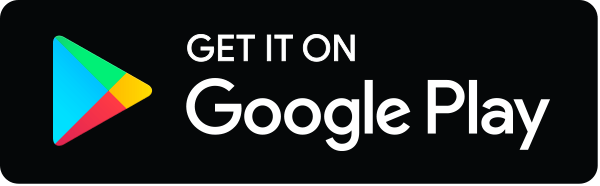
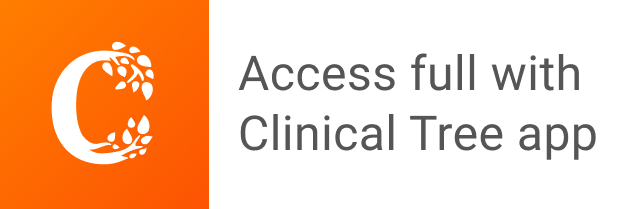