(1)
Facultad de Ciencias Médicas, Pontificia Universidad Católica Argentina, Buenos Aires, Capital Federal, Argentina
Abstract
As the Earth rotates on its axis, it has two distinct environments: light and darkness. As the axis of rotation of the Earth is tilted, the relative duration of the periods of light and darkness changes systematically during the year. Because of the process of evolution, living beings have responded to these two situations by developing specific mechanisms for prediction and have successfully adapted to the time of day and the seasons of the year. The biological rhythms are the basis of predictive homeostasis. This Chapter describes the sleep/wake cycle as the major 24-hour rhythm as well as the components of the three different ANS physiological programs that occur during it. The significance of the glymphatic system and its link to sleep are discussed.
Keywords
Clock genesDreamingγ-Aminobutyric acidGlymphatic systemMelatoninNon-REM sleepOrexinPhase mapsPolysomnographyREM sleepSeasonalitySuprachiasmatic nucleusVentrolateral preoptic areaZeitgeberObjectives
After studying this chapter, you should be able to:
Explain why the biological rhythms are the basis of predictive homeostasis.
Summarize the behavioral and electroencephalographic (EEG) characteristics of each of the stages of NREM and REM sleep and the mechanisms responsible.
Describe the pattern of normal night-time sleep in adults and the variations in this pattern from birth to old age.
Describe the interplay between brainstem neurons that contain norepinephrine (NE), serotonin (5-HT), and ACh in addition to γ-aminobutyric acid (GABA) and histamine (His) in mediating transitions between sleep and wakefulness.
Discuss the sleep/wake cycle as the major 24-h rhythm and the role of the suprachiasmatic nuclei (SCN) in its regulation.
Explain the components of the three different ANS physiological programs (“body configurations”) that occur in a normal 24-h day/night cycle.
Explain the meaning and mechanisms of dreaming.
Describe the significance of the glymphatic system and its link to sleep.
Biological Rhythms Are the Basis of Predictive Homeostasis
As the Earth rotates on its axis, it has two distinct environments: light and darkness. As the axis of rotation of the Earth is tilted, the relative duration of the periods of light and darkness changes systematically during the year. Because of the process of evolution, living beings have responded to these two situations by developing specific mechanisms for prediction and have successfully adapted to the time of day and the seasons of the year.
The brain pacemaker creates a “day” and “night” in the body , as an approximate mirror of the outside world. We wake up every day at about the same time, relatively independently of the previous time devoted to sleep. We have a greater tendency to carry out certain tasks (physical or mental) at certain times of the day or night depending on what chronotype we have (an early chronotype, “larks,” a late chronotype, “owls,” or an intermediate one).
We perceive the seasons in our emotionality, physical strength, or ability to lose weight. The disturbances originating in a traveler of transmeridian flights, the emotional imbalances that often accompany the onset of winter and the troubles experienced by those workers who must comply with rotating shifts are proof of the existence of biological clocks and calendars, in conjunction with the geophysical cycles.
Although every physiological response exhibits a 24-h rhythm, there are differences between these rhythms at the time when a peak occurs. The “phase maps ” are the graphic description of these maxima for many physiological periodic changes (Fig. 2.1). Such a sequence and spacing of the maximum values of daily rhythms reveal the ordered cause–effect relationships in bodily processes of all kinds, from genomic to behavioral, and their normality is what we could define as the quintessence of health [1].


Fig. 2.1
Phase maps of various circadian rhythms in man. As the individual is in normal light–dark conditions, each of the rhythms shown is a period of exactly 24 h. What varies for each one of them is the time in which the daily maximum (or “acrophase”) of the rhythm, shown in the diagram) is presented. A synchronized phase map characterizes normality. Reproduced with permission from Cardinali [1]
These maps may experience temporary disruptions when the body is forced to make a quick adjustment phase, as happens after a transmeridian flight [2]. In such circumstances, the different rhythmic functions do not resynchronize with the same speed and the normal temporal relationships between phases are lost. Full resynchronization requires a few days (about 1 day for every hour of phase shift), and during this period the “jet-lag” syndrome is seen. Phase maps are also distorted in chronic or acute diseases, even mild ones [3, 4]. As we discuss in Chap. 8, full recovery is achieved by controlling for both the underlying disease and the accompanying chronobiological disruption.
It is now established that clock mechanisms are genomic. Since life originated about 4,500 million years ago, in an environment where day and night already existed, successful species reproduced in their genome such geophysical reality. Thus, the day and night have left an indelible mark, which is as universal as the genetic code in all forms of life. In every living cell, a cyclic mechanism of interaction between transcription factors, genes, and proteins exists close to 24-h periods (in humans, slightly longer than 24 h; Fig. 2.2).


Fig. 2.2
Simplified model of the circadian intracellular mechanisms. The process begins when the CLOCK and BMAL1 transcription factors are dimerized and trigger the transcription of the Per (Per1, Per2, and Per3) and Cry (Cry1 and Cry2) genes. Per and Cry are translated into their respective proteins, which increase throughout the day. When the PER and CRY proteins reach a certain level, they form heterodimers that enter the nucleus and regulate the CLOCK-mediated BMAL1 transcription of their own genes. This process takes about 24 h. There are also accessory mechanisms to the clock (e.g., Rev.-ERBA and RORA). Almost 50% of the transcriptome shows a 24-h variation
These intertwined feedback loops involve a small number of clock genes [5]. The positive arm of the daily clock consists of transcription factors Bmal1 and Clock. The protein products of these genes form heterodimeric complexes that control the transcription of other clock genes, in particular, Per (Per1, Per2, Per3) and cryptochrome (Cry1, Cry2), which in turn provide the negative signal feedback that inhibits Bmal1 and Clock to complete the circadian cycle. Other clock genes (Rev-erbα, Rorα, NR1D1, timeless) provide additional force to the translation/transcription loops. The expression of clock genes is cyclic and is regulated in part by phosphorylation of proteins, thus controlling protein stability, nuclear reentry, and transcription complex formation (Fig. 2.2). Collectively, the molecular circadian clock operates in every cell of the body to regulate at least half the genome [6].
To generate physiological and behavioral responses consistently, the phases of these myriads of cellular clocks must be orchestrated by a pacemaker. One of paramount importance resides in the SCN of the anterior hypothalamus [7]. This core master clock is a key regulator of 24-h cycles in many body functions, including sleep and wakefulness, thermoregulation, glucose homeostasis, and fat metabolism [8]. SCN integrity is necessary for the generation and maintenance of the 24-h rhythms, and for their synchronization by light–dark cycles. Although the complex behaviors such as sleep, waking or feeding involve many brain areas working in a network, in the case of circadian rhythms, the participating brain region is single and has minimal volume.
The circadian apparatus includes [7]: (a) a hypothalamic pacemaker, the SCN; (b) a series of physiological outputs under the control of SCN; (c) molecular clocks present in cells of all tissues and organs. The SCN has hypothalamic (endocrine) and extrahypothalamic projections of a behavioral type. The circadian effects of SCN are exerted: (a) on neuroendocrine neurons of the hypothalamic paraventricular nucleus (PVN); (b) on autonomic PVN neurons; (c) on hypothalamic structures associated with sleep generation (e.g., the ventrolateral preoptic area, VLPO); (d) other hypothalamic areas (sub-PVN zone, sPVz; dorsomedial hypothalamus, DMH; median preoptic area, MnPO), intermediate between the SCN and autonomic and neuroendocrine neurons; on extrahypothalamic structures (lateral geniculate body, paraventricular thalamic nucleus) for the synchronization of hypothalamic conducts and locomotor activity (Fig. 2.3) [9].


Fig. 2.3
Transmission of circadian information from the oscillator to the hypothalamic systems that control circadian rhythms, including the sleep–wake rhythm. The key steps identified include multisynaptic transmission from the suprachiasmatic nuclei (SCN) to the hypothalamic control systems through adjacent nuclei of the anterior hypothalamus, multisynaptic transmission to the pineal gland controlling the secretion of melatonin , direct SCN pathways to the sleep-promoting and awakening regions, and the integration of reactive and predictive homeostasis in relation to the sleep–wake rhythm in the medial preoptic area. Modified with permission from Cardinali [8]
The SCN contains local projection neurons that communicate with one another and with other hypothalamic structures [10]. The axons of many SCN neurons terminate within the nucleus itself, thus forming local circuit connections and/or collaterals from longer range projections. The SCN core projects densely to the SCN shell, which projects only sparsely back to the core. Neuronal cell bodies in the SCN are small (~10 μm), have simple dendritic arbors, and are closely apposed.
Neurons in the SCN core and shell regions are distinguished by their neurochemical content. The neuropeptide vasoactive intestinal peptide (VIP) is found in about 10% of all SCN cells, whereas AVP is present in 20% of all cells [11]. The VIP-positive neurons are mainly located in the ventral and central parts of the SCN (core). In humans, the volume of the VIP core subdivision is 0.03 mm3 and contains about 1,700 VIP-immunoreactive neurons, with a mean density of about 63,000 neurons/mm3 [12]. Besides neurons containing VIP, substance P, gastrin-releasing peptide (GRP), calretinin- and calbindin-containing neurons are also found in the SCN core (Fig. 2.4). Most of the AVP-positive neurons are in the dorsomedial part of the SCN (the shell). In humans, the volume of the AVP subdivision is 0.2 mm3 and contains about 6,900 AVP-immunoreactive neurons, with a mean density of 29,000 neurons/mm3. In this region, neurons containing cholecystokinin (CCK) and prokineticin 2 are found in addition to AVP neurons (Fig. 2.4).


Fig. 2.4
Suprachiasmatic nuclei organization illustrating the compartmentalization of inputs and cell types and in a coronal plane. On the left, inputs originating from melanopsin-containing retinal ganglion cells (Glu; PACAP, pituitary adenylate cyclase-activating polypeptide), intergeniculate leaflet of the thalamus (NPY, neuropeptide Y; GABA) and dorsal raphe (5-HT). On the right the different cell populations described, containing AVP, CCK cholecystokinin, PK2 prokineticin 2; VIP; SP substance P, GRP gastrin-releasing peptide, CALB calbindin, CALR calretinin. 3V third ventricle, OC optic chiasm. The figure was prepared in part using image vectors from Servier Medical Art (www.servier.com), licensed under the Creative Commons Attribution 3.0 Unported License (http://creativecommons.org/license/by/3.0/)
In most SCN neurons , neuropeptides are colocalized with GABA, and almost all synapses among SCN neurons are GABAergic. Electrophysiologically, it has been shown that glutamate (Glu) is also a transmitter in the efferent pathways of the SCN.
The increased electrical activity of SCN during the day occurs in both nocturnal mammals such as the hamster or the rat and in daytime species such as the primates. In primates, however, the secretion of corticosteroids and the onset of activity and phase of sympathetic predominance and temperature rise occur at the beginning of the light phase, and not during the dark phase, as in the rat. That is, the signal produced by the SCN on the different effectors mentioned is interpreted in different ways in diurnal and nocturnal species.
Research into animals and humans has shown that only a few key environmental periodic clues, relevantly the light–dark cycle, are effective at synchronizing the internal clocks (with periods slightly longer than 24 h) to exactly 24 h [2]. A synchronizer agent can “reset” or modify the phase of the biological clock (Zeitgeber, time-giver in German). The variability of the response is always predictable, depending on when the synchronizer stimulus is applied, circadian rhythms are phase-advanced, phase-delayed, or remain unchanged (Fig. 2.5).


Fig. 2.5
Central body temperature response to the application of a light pulse or administration of melatonin (3 mg). A pulse of light during the evening or the early part of the night, slows the clock and sleep begins later on subsequent days. A light pulse during the second half of the night and early morning advances the clock and sleep begins earlier on subsequent days. Melatonin has the opposite effect. Neither light nor melatonin changed the clock if applied during the day (dotted line). Reproduced with permission from Cardinali [1]
Without the action of external time cues, the period of these oscillators tends to be longer than 24 h (Fig. 2.6). The rate is set at exactly 24 h by the action of light, which is the main (although not the unique) Zeitgeber in humans. Brief exposures to morning light are sufficient to adjust the clock to the precise 24-h solar time. This action requires an intact SCN (Fig. 2.6).


Fig. 2.6
Locomotor activity rhythm in the hamster . The activity on successive days (blue bars) coincides with the dark phase in the synchronized situation. If a few hours’ delay is made at the beginning of the night, the animal adapts with a delay (equivalent to “jet-lag”). In permanent darkness, the locomotor activity rhythm follows the endogenous period (greater than 24 h). After SCN lesion, an abolition of rhythm is observed. Modified with permission from Cardinali [8]
A group of ganglion cells located in the periphery of the retina and containing melanopsin as a photopigment projects to the SCN and other hypothalamic areas and is linked with the neuroendocrine response to light observed in all vertebrates including man [13]. They do this through specific neural projections (the retinal–hypothalamic tract), resulting in genomic activation of neurons in the SCN (Fig. 2.7). The information generated in these nuclei is transmitted to specific areas of the basal hypothalamus, which control the two major channels of body communication: the endocrine system and the ANS.


Fig. 2.7
The mechanisms triggering and maintaining sleep are in the hypothalamus and basal forebrain. The ultradian rhythm slow-wave sleep/REM sleep depends on mechanisms of the brainstem. Melatonin has effects on both central and peripheral oscillators. Reproduced with permission from Cardinali [1]
In humans, light during the first part of the night delays the clock and that during the second part of the night and early morning accelerates the clock (Fig. 2.5). At other times of the day, exposure to light exerts no appreciable effect to advance or delay the phase of the circadian rhythms. This mechanism explains why exposure to artificial light fastens the endogenous clock in the morning, whereas during the first part of the night it tends to perpetuate and aggravate sleep deprivation by causing a phase delay [2].
A major synchronizer of the SCN clockwork is pineal melatonin (Figs. 2.7 and 2.8) [1]. Melatonin synchronizes the human circadian system as per a phase response curve that is about 12-h out of phase with the phase response curve produced by light (Chap. 8). Projections of the SCN driving the daily melatonin rhythm inhibit the firing of neurons in the sPVz of the hypothalamus. From this zone, a multisynaptic pathway begins that includes the medial forebrain bundle, reticular formation, and the intermediolateral cell column of the cervical spinal cord, the superior cervical ganglia (SCG), and the postganglionic sympathetic fibers that end near the pineal cells to stimulate melatonin synthesis (Figs. 2.7 and 2.8).


Fig. 2.8
Control of melatonin synthesis by environmental light. Retinal ganglion cell receptors project via the retino-hypothalamic tract (RHT) to the SCN. From here, and through a multisynaptic pathway including the cervical sympathetics, pineal melatonin release is modulated. Melatonin both feedback at the SCN and affects peripheral clocks. Reproduced with permission from Cardinali [1]
Melatonin phase-shifts circadian rhythms in the SCN by acting on MT1 and MT2 melatonin receptors in SCN neurons [14]. The phase-and amplitude-altering effect of melatonin is caused by its direct influence on the electrical and the metabolic activity of the SCN. The circadian rhythm of melatonin secretion has been shown to be responsible for sleep rhythm in both normal and blind subjects (i.e., in the absence of the synchronizing effect of light). If an individual who usually falls asleep after midnight wishes to advance his or her sleep schedule to rise early for work, the indication is the administration of melatonin at 18:00–19:00 h to achieve the desired phase advance of the circadian clock (Fig. 2.5) [1].
The SCN communicates day–night cycle information to the rest of the body through neural and humoral signals, including the ANS and the neuroendocrine system [7]. By this information, the peripheral cellular circadian clocks become synchronized to exactly 24 h. As is discussed in Chap. 4, the clocks at the periphery are also able to respond to other environmental cues, such as food, altering their phases to these cues accordingly.
Both melatonin and cortisol secretion are controlled by the circadian clock and in turn are essential for synchronizing peripheral circadian rhythms. The secretion of melatonin is very consistent from day to day for a given period of life and shows far fewer contingent changes because of the stress that cortisol shows. Thus, melatonin reflects more accurately the circadian signal given by the SCN [1].
From an evolutionary point of view, it is not difficult to imagine that a successful adaptation to the environment in which animals compete for nutrients that are always scarce needs to optimize processes of high-energy consumption, such as reproduction [15]. Thus, almost all species undertake seasonal mating in the wild (Fig. 2.9). The most appropriate signal to the circadian system to transmit information about the season is the duration of photoperiod. Other environmental signals (temperature, humidity, etc.) do not have the same degree of reproducibility year after year as the length of the photoperiod.


Fig. 2.9
Reproductive seasonal rhythm in the sheep (a short-day breeder). Modified from Cardinali [8]
In humans, there is seasonality in reproduction. The statistics on births indicate the seasonality in the reproductive process, with maximum activity during the summer. If multiple births are computed, which are indicative of ovarian overstimulation and are therefore independent of social factors such as sex behavior, the seasonal differences are even more significant. These studies have been conducted in populations in the northern hemisphere in periarctic zones (Scandinavia, Labrador Peninsula). In these countries, the activity of the pituitary–ovarian axis and the incidence of conception in human populations decline during the dark months of the year.
In comparative studies between summer and winter conducted in Finland, increased melatonin secretion was observed during the winter, coinciding with the decline of ovarian hormone release [16]. The overnight pulse of melatonin in the plasma extends for a few hours in the morning during the winter because of the short duration of the day (3–4 h) and low light intensity caused by the very oblique incidence at a latitude of 65° north, light intensity being insufficient to suppress melatonin secretion. This may be the signal that triggers gonadal involution. Menarche has a seasonal incidence, with peaks in the spring and summer. Seasonality in the reproductive process also occurs in men [17].
Long day breeders such as rodents, with a gestational period of less than a month, mate at a time that allows the pups to be born at a time of the year that maximizes survival (the summer). In a long breeder rodent such as the rat kept under optimal light conditions and laboratory feeding, the seasonal changes tended to disappear. However, it can be restored by the administration of melatonin in the drinking water in a form that resembles a photoperiod exposure to winter [18].
In short day breeders such as sheep or deer, mating takes place in the autumn, when nights lengthen and prolonged levels of melatonin secretion occur (Fig. 2.9). This is because the gestational period in this species is longer (5 months); thus, the birth of the pups occurs in the period when chances of survival are likely to be greatest (spring). In these animals, melatonin stimulates reproductive function by decreasing the sensitivity of the hypothalamus to the negative feedback of gonadal steroids.
Another seasonal rhythm is that of mood [19]. Although seasonal trends of mood and emotions have been recognized in the medical literature for over 100 years, it was not until the 1980s that the distinguishing features of an affective disease involving the recurrent winter depression were established. This form of affective illness (“seasonal affective disorder”) is now the subject of numerous investigations [19]. Every fall or winter, patients with this condition get tired easily, eat high-calorie carbohydrate diets, show weight increases, and have exaggerated anxiety or sadness. With the arrival of spring, patients emerge out of depression, and in certain circumstances, may show moderate manic symptoms.
Light therapy is useful in seasonal affective illness: the morning exposure to light (intensity of at least 2,500 lux, equivalent to the light intensity of a sunset) for 2 h daily is recommended [19].
One could see seasonal affective disorder as the human equivalent of hibernation. Decreased libido in the winter reduces the chances of birth the following winter (an inappropriate time of the year for newborn survival). In addition, food intake increase in the winter facilitates reproductive success because overweight mothers tend to have larger fetuses. In the winter, at high latitudes, nocturnal melatonin secretion in humans is about 40 min longer than in the summer [16]. In a hibernating animal species, the prolongation of 30 min in the secretion of melatonin is sufficient to signal winter.
Evidence has now accumulated that a seasonal change in thyroid hormone availability within the brain is a crucial element for seasonality [15]. This is mediated by local control of thyroid hormone metabolizing enzymes within ependymal cells lining the third ventricle of the hypothalamus. Within these cells, deiodinase type 2 enzyme is activated in response to the length of summer days, converting metabolically inactive thyroxine to tri-iodothyronine. The pars tuberalis of the pituitary gland plays an essential role. Specialized thyrotroph cells are regulated by the changing signal of day length, with long days activating TSH. In mammals, the pars tuberalis is regulated by the nocturnal melatonin signal [15].
The Sleep/Wake Cycle as the Major 24-h Rhythm
A fundamental circadian rhythm is that of sleep/wakefulness. The Greeks called sleep “the brother of death,” because they thought that in the sleeping man, the soul temporarily abandoned the body and wandered through the world. Indeed, sleep is an active process, the metabolism of several brain regions being greater than in wakefulness. Moreover, sleep is complex, with two different electroencephalographically (EEG) defined stages, NREM and REM sleep. It is also endogenous and relatively independent of exogenous factors. During REM sleep , O2 consumption in various brain areas of the limbic system exceeds that found in wakefulness [20].
In rats, total sleep deprivation (NREM plus REM sleep) causes death in about 20 days. Hair loss and discoloration, tail and leg skin lesions and increased food intake, together with 20% body weight loss and a doubling of energy expenditure, are seen in sleep-deprived rats. If the deprivation is only of REM sleep , rats die in about 40 days, with the same abnormalities. On average, humans cannot live without sleep for more than a few days (about 2 or 3 days). After sleep deprivation, humans recover approximately 33% of the total sleep time, 100% of the slow-wave sleep, and 30–50% of the REM sleep lost [20].
A reduction of daily sleep hours is common in contemporary society, which prioritizes a timeless organization, with continuous activity 24 h, 7 days a week (“24/7 society,” Chap. 8). On average, we sleep about 2 h less every day than just 40 years ago, and this affects our cognitive performance, emotional stability, and health [1].
Electroencephalography recording and ocular musculature activity during sleep has allowed the study of the “basic architecture” of the neural process. Four stages of sleep are distinguished according to the type of EEG brain activity (Fig. 2.10). Stages N1–N3 correspond to the progressive slowing of the brain waves, from the α rhythm (8–13 Hz) to the δ rhythm (<3 Hz). A fourth stage, REM sleep, corresponds to a desynchronized EEG, similar to that of wakefulness [20].


Fig. 2.10
Sleep stages based on electroencephalography (EEG). There is a slowing of the brain waves (N1–N3 stage) depending on the progression of sleep, reaching deep slow-wave sleep in about 30–45 min. At this time, a progressive acceleration of EEG occurs, running from stage N3 to N1 in about 30–45 min to reach the REM sleep stage (R), which lasts about 10–15 min. There are between 4 and 6 cycles per night. Reproduced with permission from Cardinali [1]
Rapid eye movement (REM) sleep is accompanied by a profound loss of muscle tone (except for the diaphragm, the muscles of the middle ear, and the cricoesophageal sphincter, all of which have very few muscle spindles), ponto-geniculo-occipital (PGO) spikes on the EEG, eye movements synchronous with PGO spikes, and ANS signs, such as increased variability in BP and heart rate and the tendency toward poikilothermia (loss of control of body temperature). The more complex mechanisms of cardiocirculatory, respiratory, and thermal feedback temporarily cease to function during REM sleep, with only the autonomic spinal reflexes remaining.
The normal polysomnographic register in humans indicates a slowing of the cerebral waves (stages N1–N3). An acceleration of the EEG rhythm then occurs, passing from stage N3–N1 in about 30–45 min (Fig. 2.10). Abruptly, a stage of REM sleep occurs that lasts about 10–15 min, beginning a new cycle of slowdown and subsequent acceleration of brain waves (Fig. 2.10). There are four to six REM sleep episodes per night, but a δ rhythm (N3 stage) is only detected during the first half of the night. In the second part of the night, the inter-REM periods show increased EEG frequency, but not passing the stage 2 or 3 in the final hours of sleep. In contrast, the REM periods are longer in the second part of the night. On average, normal adult polysomnographic recordings demonstrate 25% REM sleep and 75% NREM sleep (slow-wave sleep; 50% in stages N1 and N2, and 25% in N3). Video recordings detect a postural change of importance every 20 min, approximately, all of them corresponding to NREM sleep.
Three mechanisms have been identified as responsible for sleep (Fig. 2.11) [21]:

A process called “S” (for sleep), determined by the previous individual history of sleep and wakefulness. The “S process” is manifested in the increased propensity to sleep after sleep deprivation. It is the accumulation of sleep debt, like the mechanism of an hourglass.
A process called “C” (for circadian), controlled by the endogenous biological clock. It is independent of the previous history of sleep and wakefulness. The “C process” comprises the trend toward falling sleep with the decrease in body temperature (during the first part of the night) and the termination of sleep during the increase in body temperature (during the second part of the night). This is because of the “C process,” that is, after a night spent awake one is sleepier at 04:00–05:00 h than at 07:00–08:00 h, regardless of the 2–3 h increase in sleep debt. Therefore, sleep is like a bank debt: it is impossible to pay the debt when the “bank window” (C process) is “closed.” For a night worker who after a sleepless night wants to sleep in the morning, he must wait until a more appropriate time (e.g., the siesta after lunch) to have a restful sleep.
An ultradian component (frequency of about 90 min), perceptible both in sleep (slow-wave sleep and REM sleep alternation) and in wakefulness (periodicity of about 90 min with maximal and minimal attention, the so-called basic rest–activity cycle, BRAC) [22].

Fig. 2.11
Three interacting processes regulate the timing, duration, and depth, or intensity, of sleep: a homeostatic process that maintains the duration and intensity of sleep, a circadian rhythm that determines the timing of sleep, and an ultradian rhythm given by the NREM sleep–REM sleep sequence. Reproduced with permission from Cardinali [1]
It is important to note that these three mechanisms apply not only to sleep, but also to the ultradian and circadian variations of most of the physiological phenomena in which they have been examined. Studies conducted in sighted individuals under normal sleep–wake cycles cannot establish whether the rhythm is endogenous and self-sustained (the definition of a circadian rhythm) or is driven by external factors such as sleep–wake or rest–activity cycles, the light–dark cycle, or feeding cycles. The constant routine protocol is a gold-standard method employed in circadian biology to differentiate whether or not a rhythm is intrinsically generated and sustained and, compared with measures taken under ambulatory baseline conditions, can measure the extent to which it is driven by external factors such as sleep, light, meal timing, or posture [2]. During a constant routine procedure, participants remain awake in bed for typically 30–50 h (1–2 circadian cycles) in a semirecumbent posture under dim light conditions and are fed hourly isocaloric meals. This procedure removes the direct impact of sleep, light, activity, and posture on rhythm expression and distributes calorie intake uniformly across the circadian cycle, hence removing or minimizing many invoked effects due to external factors that may mask the underlying endogenous circadian rhythm. An important point to consider in relation to the ultradian/circadian relation is that the ultradian period of 90 min is a harmonic of 24 h and that its progressive consolidation (rhythms of 1.5 h, 3 h, 6 h, 12 h, 24 h) is seen as the ontogenic development of the sleep/wake rhythm in human newborns.
The C process involves circadian changes in the promotion of wakefulness given by the SCN (Fig. 2.12) [7]. In primates that have lesions in the SCN, not only the desynchronization of circadian rhythms occurs, but a significant increase in the total time asleep (having removed a key area for maintaining wakefulness) is seen (Fig. 2.6). During the day, the electrical activity of the SCN increases and peaks toward the evening (around 18:00 h). SCN neurons help to counteract the increased pressure of sleep debt that accumulates during wakefulness [7].
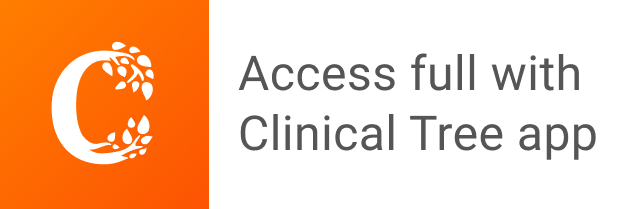