Abstract
The dorsal root ganglion (DRG) is a unique neural structure in the nervous system as it not only houses the somata of the primary sensory neurons (PSNs) but also is critically important in the development and maintenance of chronic pain. Recent technological advances have made it possible to effectively deliver neuromodulation therapy to this structure for the treatment of chronic pain. Specific neuroanatomic and physiologic aspects to the somatic nervous system and the DRG provide insight as to why this therapy can be so particularly advantageous for certain chronic pain conditions. Multiple mechanisms of action have been studied and include direct effects of the electrical field on PSNs in the ganglia, as well as indirect effects on upstream and downstream neural elements within the neuraxis. Both a systems and cellular approach are taken to understand this therapy and what potential advantages it may provide chronic pain patients.
Keywords
Dorsal root ganglion, DRG, Mechanism of action, MOA, Neuromodulation, Physiology
Authorship Statement
All authors made a substantial contribution to the study’s concept and design and approved the final version of the manuscript.
Conflict of Interest Statement
Drs. Kramer, Kent, and Cusack are employees of St Jude Medical.
Introduction
Electrical neuromodulation for the relief of chronic pain has a long and colorful history, beginning with the often-repeated anecdotes involving electric fish ( ), spanning galvanic applications of somewhat dubious merit ( ), and encompassing a number of reports of do-it-yourself approaches in the present day ( ). However, on the peer-reviewed publication of prospective investigations ( ) and the contemporaneous development of an explanatory mechanistic model based on a modern understanding of neurophysiology ( ), neuromodulation has entered the contemporary era.
For more than 50 years, the neural–technology interface has been refined. Today, dozens of hardware designs exist for pain management via neuromodulation, and thousands of patients have experienced meaningful improvements of their symptoms, function, and quality of life (QOL). Spinal cord stimulation (SCS) is the most widely used of the implanted neuromodulation options and is typically used for neuropathic pain of the trunk and/or limbs. Traditionally, for SCS, electrical contacts are placed epidurally above the dorsal columns (DCs) of the spinal cord. Two styles of leads exist: (1) cylindrical linear leads that are maneuvered to their epidural sites through an epidural needle/catheter in a percutaneous procedure under fluoroscopic guidance and (2) unidirectional paddle-shaped leads that are placed via open laminotomy or laminectomy. The former is minimally invasive and can be performed by anesthesiologists or other nonsurgical specialists, while the latter requires neurosurgical or orthopedic involvement.
A recent systematic review and meta-analysis calculated that the average pain relief with SCS was 58% ( ), and measures of QOL, function, and sleep likewise improve ( ). In accordance with the International Classification of Functioning, Disability, and Health (ICF model), this makes it an extremely valuable intervention for otherwise-intractable cases. Currently, more than 30,000 Americans receive SCS systems each year ( ). Despite its undisputed benefit, SCS does not meet the needs of all people with chronic neuropathic pain for various reasons:
- 1.
Pain relief may be insufficient. Adequate response to SCS is generally defined as relief of 50% or more of baseline pain as measured on a standard visual analog scale (VAS) or numeric rating scale (NRS), although this is only a rule of thumb; the minimal clinically important difference for back pain is 2–3 cm on a 10-cm VAS ( ). About 20% of patients do not respond to initial treatment during the temporary trial, and about 25% of those implanted do not respond long term ( ). Thus, a substantial proportion of patients, having already exhausted conventional pain management interventions, are not served by SCS either.
- 2.
Pain in certain regions may not be amenable to treatment. Pain–paresthesia concordance, known to be a necessary condition for achieving pain relief of the painful trunk and legs, but not angina pain, with traditional tonic SCS ( ), is readily achieved for the limbs but is more difficult for trunk sites. This is likely due to an inability to recruit the necessary DC fibers as a result of spinal anatomy and electrode location ( ). This may result in SCS patients having residual pain (e.g., patients may report that SCS relieves their leg pain but not their back pain) or patients being deemed unsuitable for SCS treatment in the first place due to the site of their pain.
- 3.
Treatment is highly dependent on lead location. Because there is a strong relationship between dermatomal coverage and spinal level, there is a narrow rostrocaudal range within which lead placement is appropriate and efficacious ( ). Midline versus lateral lead placement is also relevant ( ). Patients with epidural adhesions or other spinal abnormalities may not be good SCS candidates due to potential complications during implantation.
- 4.
Side effects may be intolerable. Some SCS patients report extraneous paresthesias in nonpainful regions, abrupt changes in the perceived intensity of stimulation with changes in position ( ), and/or uncomfortable or “segmental” stimulation, relating to the preferential recruitment of collaterals ( ). These side effects may limit the effective usage range of SCS.
- 5.
Lead migrations are a common complication (although, certainly, not the only one; ). Migration rates have been reported at 8.5% ( ), 13.2% ( ), and 15.5% ( ) in comprehensive reviews of the literature. Migrations are most often detected due to a complaint of loss of therapy or movement of paresthesia to unwanted areas and generally require surgical resolution.
- 6.
Some SCS programs can have high power consumption. In primary cell devices (nonrechargeable battery), surgical procedures for battery replacement are needed every 3–4 years ( ). In rechargeable devices, frequent recharging is necessary ( ).
DRG stimulation was developed in response to these unmet needs and challenges related to conventional SCS. Some preliminary work investigated the DRG as a target for neuromodulation ( ) but did not progress due to the technical difficulty of introducing standard percutaneous leads into the vertebral foramen. The development of specialized technology has now made DRG stimulation feasible. The sections below describe the current knowledge base for this intervention.
The DRG as a Target for Neuromodulation
DRGs are bilateral structures located on the dorsal root of the spinal nerve at each spinal level ( ) ( Fig. 16.1 ). Embryologically, DRGs are descended from progenitor cell lines in common with the spinal cord ( ), and because they are enclosed in the dural sac, DRGs are part of the central nervous system. However, their physical location, which is separate from the spinal cord and segregated within the vertebral foramen, places them in a unique position at the junction with the peripheral nervous system. Higher permeability of the blood–brain barrier at this site suggests that these structures may have an as-yet-undefined chemosensory role ( ).

Sensory receptors and the afferent fibers of PSNs are densely arborized throughout the periphery. Fibers of individual PSNs coalesce to form sensory nerves that traverse the dorsal roots and enter the superficial layers of the DCs of spinal cord ( ). PSN somata are located in the DRGs; cell bodies and processes of these pseudo-unipolar neurons are linked with a T-junction process. The DRG contains two main categories of PSN somata: large, lightly staining cells (Aα and Aβ neurons) and small darkly staining cells (Aδ and C neurons; ). A large population of satellite glial cells (SGCs) and immune cells also exists in the DRG ( ). An individual DRG is likely to receive sensory information from the periphery in a roughly dermatomal fashion, although evidence is emerging that complex intersegmental convergence of DRG projections or branching of PSN dendrites exists ( ) and can create functional receptive fields that are either discrete/subdermatomal or very broad.
Action potentials (APs) are generated in the periphery in response to sensory stimuli, propagate orthodromically, and are transmitted to interneurons and wide dynamic range (WDR) neurons in the gray matter of the spinal cord. Additionally, Aα- and Aβ-fibers (the targets of SCS) ascend in the fasciculi cuneatus and gracilis to supraspinal nuclei. The somata, located in the DRG, may modulate the incoming peripheral activity before it is transmitted to the spinal cord at both the single-cell and population levels. More specifically, at the population level, electrophysiological recordings have demonstrated that subthreshold depolarizations can be detected in neighboring PSN somata ( ), either through a neurochemical communication or direct gap junction–mediated communication or through the function of the electrically active SGCs ( ).
The DRG has a role in the initiation and maintenance of neuropathic pain. Damage to nerves, either by peripheral trauma or by compression, initiates a number of biochemical cascades. In the DRG, following an injury, ligand receptor and ion channel expression is upregulated, and neurotrophins and inflammatory cytokines are released by both neurons and glia. Native macrophage, lymphocyte, and glial populations proliferate and sympathetic fibers sprout to contact PSNs ( ). Cross-excitation from one neuron to another increases ( ). Inward calcium currents and outward potassium currents are reduced, which results in attenuated afterhyperpolarizations following APs, thus increasing the likelihood of repetitive firing ( ). Additionally, upregulation of delayed-conductance, voltage-dependent sodium channels establishes oscillatory transmembrane potentials and ectopic discharges in a model of injured DRG neurons ( ). The functional consequence of these changes is a net increase in DRG activity via ectopic discharges and potentiated responses to mechanical or sensory stimulation. The proportion of spontaneously active DRG neurons can increase 8-fold under conditions of nerve injury ( ), and the number and amplitude of depolarizations in response to sensory stimuli also increase dramatically ( ). These electrophysiologic changes are associated with the experience of pain in humans ( ) and with pain behaviors such as paw withdrawal in animal models ( ). The DRG independently generates ectopic pulses ( ), which are maintained at high levels long after the acute injury has healed ( ). This is theorized to be a key driver of neuropathic pain ( ). Electrical field stimulation of in vitro DRGs reduces their firing rates ( ), which suggests, at a broad level, a mechanism of action (MOA) for DRG stimulation. Further discussion regarding MOAs is given in the next section.
The DRG stimulation system’s design (Axium; St. Jude Medical, Minneapolis, MN) is similar to that of other SCS devices; that is, it uses an implantable power generator (IPG) and up to four cylindrical leads. The IPG uses a nonrechargeable battery and constant current stimulation. The leads are narrow in diameter (1 mm) and extremely flexible and have a hollow inner lumen into which a stylet can be inserted to provide rigidity and directionality during implantation.
The implantation procedure is minimally invasive and completed with the patient under light sedation ( Fig. 16.2 ). Access to the epidural space is gained with a needle using the standard loss-of-resistance technique. The lead, with its stylet in place, is loaded into a delivery sheath and introduced into the epidural space through the needle. The leads are advanced anterograde and steered into the intervertebral foramen near the DRG target using fluoroscopic guidance. Because the DRG is located between the medial and lateral aspects of the pedicle ( ), a pair of the lead’s electrical contacts should straddle this space.

Intraoperative stimulation, with feedback from the patient regarding the location of perceived paresthesias, is completed to confirm proper lead placement. Lead slack is deployed in the epidural space as the delivery sheath is removed, and the leads are anchored against the fascia. Leads are tunneled subcutaneously to the IPG pocket. Trial periods can be used before permanent implantation by the externalization of lead extension cables and connection to an external trial stimulator.
DRG stimulation is indicated for treatment of complex regional pain syndrome (CRPS) in the United States and is used for neuropathic pain arising from a wide variety of etiologies. The patient populations for DRG stimulation are similar to those for SCS and most commonly represent CRPS, failed back surgery syndrome (FBSS), and peripheral neuropathies ( ). DRG stimulation is generally considered for chronic neuropathic pain of moderate/severe intensity after conservative options have failed (but without delay because it is presumed that, as in SCS ( ), earlier intervention is associated with better outcomes). Patients who have a surgically remediable source of pain, major comorbidities including psychiatric instability and coagulopathies, and other contraindications to surgery are poor candidates. A trial period of up to 30 days is generally advisable; a successful trial outcome is generally one in which results are 50% or better relief from baseline pain ratings ( ).
The paresthesia patterns that can be delivered with DRG stimulation differ from those of SCS. Although DRG stimulation is capable of providing broad, full-limb coverage for radicular pain (similar to SCS), more often it is perceived in discrete, subdermatomal distributions and can be as specific as providing coverage of a single toe ( ). DRG stimulation can also readily deliver analgesia to the groin ( ) and low back, regions that can be challenging to recruit with SCS systems. For these reasons, DRG stimulation may be selected instead of SCS for patients who have focal peripheral pain or primary axial pain. Because the paresthesias produced by DRG stimulation do not vary over time or based on body position ( ), DRG stimulation leads may not be as susceptible to lead migration or positional stimulation compared with SCS. This may be due to their bony encasement inside the vertebral structures and/or the thin cerebrospinal fluid (CSF) layer between the contacts and the neural target.
Mechanisms of Pain Relief
There are a number of potential MOAs for DRG stimulation, as outlined next ( ). None logically contradict the others; in fact, it is likely that these mechanisms may overlap and complement each other ( Fig. 16.3 ).
- 1.
Antidromic activation of peripheral fibers may play a role in the mechanism of DRG stimulation by directly modulating the patterns of incoming afferent impulses ( ), for example by creating a collision block or by peripheral sensitization. This may be especially important for peripherally maintained sources of pain, such as neuromas.
- 2.
DRG stimulation is likely to reduce the rate and/or amplitude of APs arising from DRG neurons ( ), thereby correcting the hyperexcitability of sensory neurons that is characteristic of neuropathic pain. This may result in neuroplastic shifts in the ion channel landscape of DRG somata to a more normal phenotype as well as a reduction in inflammatory markers. The involvement of SGCs may also be downregulated.
- 3.
The T-junction into the DRG is thought to act as a band-pass filter for afferent PSN activity ( ) due to the resistance and delay introduced by the bifurcation point. Injury-induced changes in calcium currents in the DRG alter the filtering properties and allow more high-frequency activity to enter the central dendrites and the spinal cord ( ). DRG stimulation may restore the natural filtering threshold to more normal levels.
- 4.
Lowering the firing rates of DRG neurons via DRG stimulation changes their input to interneurons and WDR neurons in the DC of the spinal cord that likely involves GABAergic processes ( ). The removal of overactive DRG input may allow the intraspinal activity to reduce and normalize ( ).
- 5.
As a result of the changes at these sites, the communication with supraspinal sites is also likely to be modulated. Brain regions involved in the perception/modulation of pain include the periaqueductal gray, ventroposterolateral thalamus ( ), dorsal anterior cingulate cortex, and prefrontal cortex ( ), although exact pain pathways are unknown ( ).
- 6.
Broad connections exist between the DRG and sympathetic trunks ( ). In nerve injury models, the sensory-sympathetic coupling in the DRG increases ( ). Given the effectiveness of SCS for sympathetically maintained pain ( ), it seems reasonable that the mechanisms of DRG stimulation may also modulate activity along this pathway.
- 7.
Although superimposition of paresthesias with the pain appears to be a necessary component for successful DRG stimulation, the generation of perceptible paresthesias during everyday use is not; a considerable proportion of patients use DRG stimulation parameters that are below the perception threshold. Paresthesias, therefore, may simply be an epiphenomenon that that can be usefully exploited during implantation and for programming purposes.

To better understand the impact that DRG stimulation can have on the conduction of APs through the PSN, computational modeling was conducted ( ). A two-stage computational model was constructed to calculate the response of primary sensory C-neurons to DRG stimulation. The first stage was a finite element analysis (FEA) model that calculated electrical potentials generated in the neural tissue during bipolar DRG stimulation ( Fig. 16.4A ). The data generated with the FEA model were then used to populate the parameters used in the second stage, which was a biophysical cellular model of C-neurons in the DRG (the neurons that are likely to carry nociceptive sensory modalities). The transmembrane potential was measured at different locations along the cell: on the peripheral axon at a point 31.6 mm distal to the T-junction, at the T-junction itself, at the mid-point of the “stem” axon (the unitary process extending from the soma), the soma, and on the central axon at a point 37.7 mm proximal to the T-junction ( Fig. 16.4B ).

The first experiment using the computer model was intended to test the effect of DRG stimulation on low-pass filtering at the T-junction of the C-neuron. Simulated sensory stimuli were delivered to the peripheral axon at 4 Hz. Synchronized afferent traffic propagated from the origin site in the peripheral axon through the T-junction and was detected in the stem, soma, and central axon ( Fig. 16.5A ). However, when 40-Hz DRG stimulation was delivered to the C-neuron, ongoing block was generated at the T-junction such that the afferent stimuli APs did not propagate into the central axon ( Fig. 16.5B ). This filtering effect was generated via hyperpolarization of the T-junction, stem, and soma and depended on the activation of calcium-dependent K SK + channels in the soma. Thus, DRG stimulation blocked afferent traffic propagating from the periphery ( ).

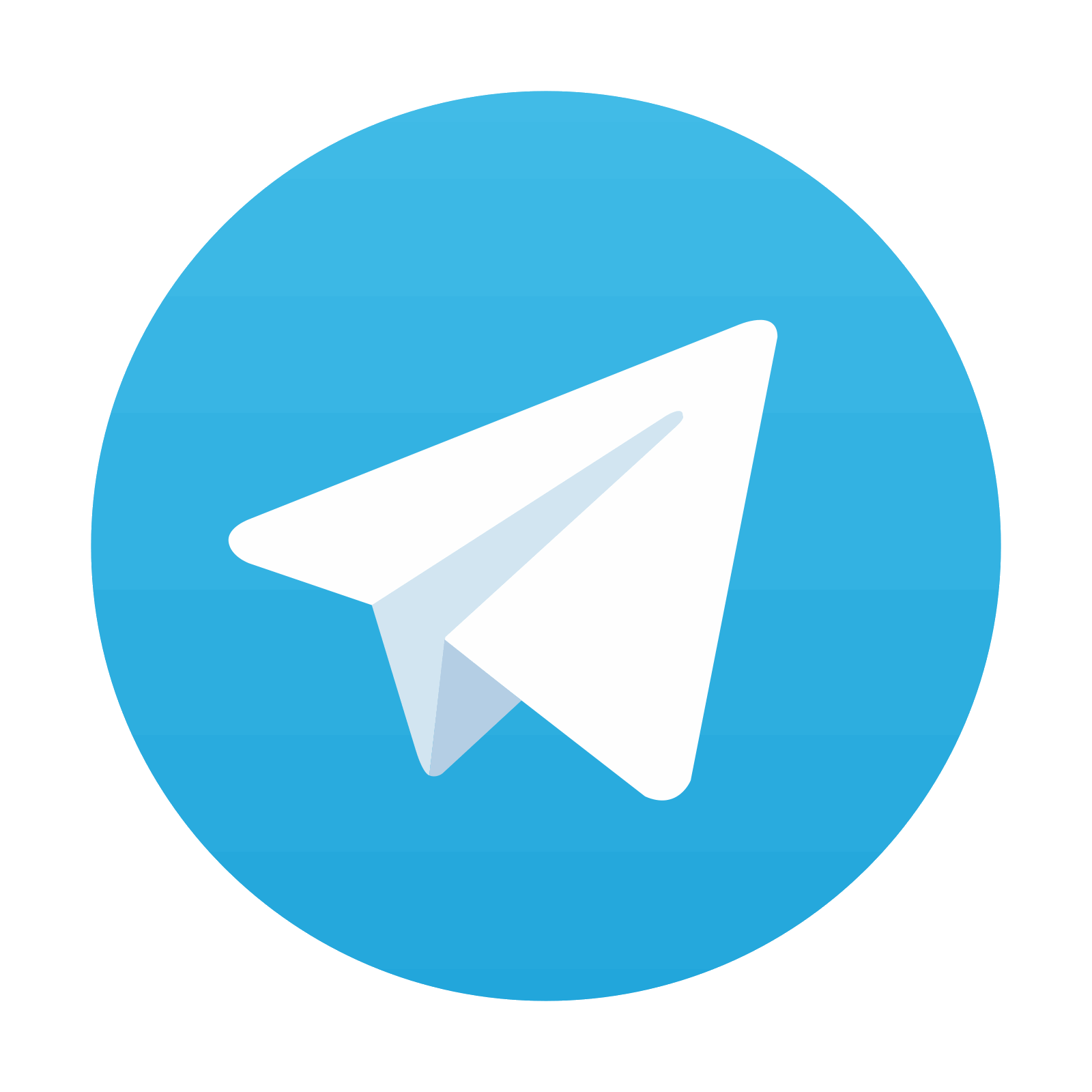
Stay updated, free articles. Join our Telegram channel
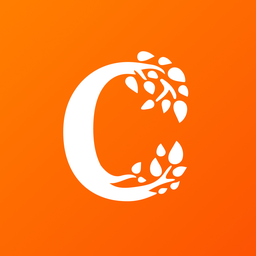
Full access? Get Clinical Tree
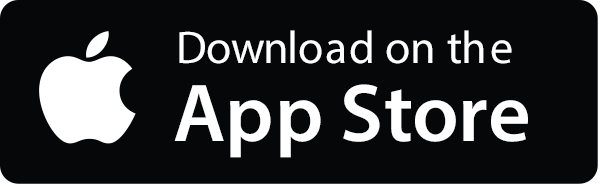
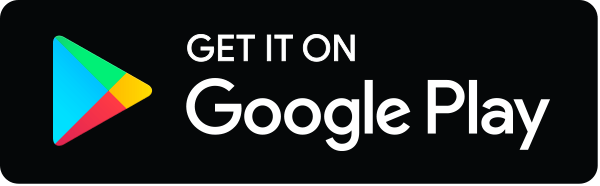
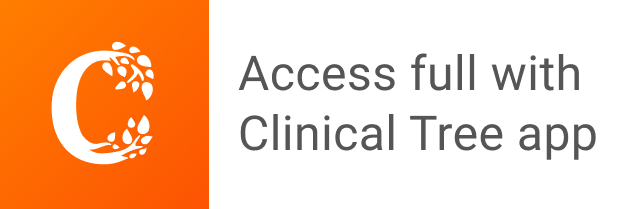