Abstract
Gene therapy, the delivery of therapeutic genes to the nervous system to restore function, represents a promising alternative approach for the treatment of neurologic disorders for which pharmacotherapy and deep brain stimulation may be inadequate. Numerous clinical trials have demonstrated not only the potential of gene-based neuromodulatory strategies to treat genetic, degenerative, and oncologic diseases of the nervous system but also their safety and feasibility in patients. Moreover, our evolving understanding of the complex neural circuitry underlying neurologic disease is leading to elucidation of novel disease targets, gene-based approaches, and methods of noninvasive gene delivery and control. Continued advances within the field promise to expand the role of gene-based neuromodulation in treating neurologic disease. Here, we review the underlying technology of central nervous system gene therapy as well as data from a variety of human central nervous system gene therapy clinical trials, and then discuss emerging innovations in gene therapy that may help broaden its applications to a wider range of neurologic and psychiatric disorders.
Keywords
Clinical trials, Gene therapy, Nervous system, Neurologic disorders, Viral vector
Outline
Introduction 429
Gene Therapy Vectors 430
Viral Vectors 430
Control of Transgene Expression 431
Human Clinical Safety and Efficacy Data for CNS Gene Therapy 432
Genetic Diseases 433
Canavan Disease 434
Batten Disease (Late Infantile Neuronal Ceroid Lipofuscinosis) 434
X-Linked Adrenoleukodystrophy 434
Ongoing Gene Therapy Considerations for Genetic Diseases of the CNS 434
Neurodegenerative Diseases 435
Brain Tumors 436
Discussion of Future Trends and Pathways to Expanding the Knowledge Base 436
Novel Disease Targets 436
Novel Modulatory Gene Therapy Approaches 437
Novel Approaches for Noninvasive Gene Delivery 438
References 439
Introduction
One billion people worldwide have a neurologic disorder ( ), and despite great demand for effective therapies, treatment options for these disorders remain quite limited. Interventions to deliver genes to the nervous system that permanently restore function are a powerful alternative to existing standard pharmacologic approaches; such approaches, in which DNA or RNA is used as the therapeutic agent, are defined as gene therapy. Though historically fraught with controversy in the early 1990s, gene therapy has reemerged over the past decade as a safe, feasible, and targeted potential therapeutic for a range of neurologic disorders. The complexity of the nervous system has long posed a challenge to scientists and physicians looking to develop efficacious treatments for diseases affecting the central nervous system (CNS). While pharmacotherapy and deep brain stimulation (DBS) are the current mainstays for treating neurologic disease, both approaches have significant limitations regarding specificity, time course, and side effect profile. Ideally, next-generation therapies for neurologic disease will be noninvasive, bidirectional, spatially precise, and temporally regulatable: in the near future, gene therapy has the very real potential to meet all of these criteria. This chapter will provide a review of the relevant history and current knowledge base of gene therapy, a summary of developments over the past decade including completed and ongoing clinical trials, and a discussion of future trends and pathways toward expanding the knowledge base.
Gene Therapy Vectors
The complexity of the nervous system and the multigenetic nature of most neurologic disorders continue to present significant challenges to the design and implementation of successful gene therapies in the clinic. The efficacy of gene therapy is dependent on effective gene transfer, and great efforts have been made since the advent of gene therapy in the mid-1980s to design vectors that efficiently deliver therapeutic genes to the nervous system to replace defective genes. The delivery of genes into cells can be accomplished by multiple methods. Nonviral gene delivery methods, such as naked nucleic acid, liposomes, and nanoparticles, while advantageous in their low cost and ability to deliver large genetic payloads, have historically resulted in low therapeutic gene expression of limited duration ( ), although recent advances are beginning to rectify this. The development of viral-based approaches, which exploit the innate ability of a virus to insert its DNA into host cells, however, has been the most promising method of gene delivery over the past two decades. To date, the primary viral vectors for CNS gene transfer are adeno-associated viral (AAV) and lentiviral (LV), although herpes simplex viral (HSV), and adenoviral (AV) vectors have also been used to treat nervous system disorders and will be touched on briefly here.
Viral Vectors
As mentioned, the hijacking of viral biology for transgene expression rather than viral replication within host cells is key to the development of an efficacious viral vector for gene therapy. Importantly, each of these viral vectors has advantages and disadvantages for specific gene transfer needs as they vary considerably with respect to transgene insert size, duration of transgene expression, cellular target, and degree of immunogenicity. Table 30.1 depicts the characteristics of the most commonly used viral vectors. The design of any viral-based gene therapy strategy, therefore, should take into account the inherent technical strengths and limitations particular to a given viral vector.
Adeno-Associated Virus | Lentivirus | Herpes Simplex Virus | Adenovirus | |
---|---|---|---|---|
Genome | Single-stranded DNA | Single-stranded RNA | Double-stranded DNA | Double-stranded DNA |
Transgene capacity | ∼4.5 kb | ∼8 kb | ∼40–50 kb | ∼7.5 kb |
Host genome integration | No | Yes | No | No |
Neuron targeting | Yes | Yes | Yes | Yes |
Onset of transgene expression | Slow | Fast | Fast | Fast |
Expression duration | Long | Long | Short | Short |
Number of serotypes | >100 | 5 | 2 | ∼50 |
Typical gene delivery method | CNS in vivo | Ex vivo transfection of hematopoietic stem cells | CNS in vivo | CNS in vivo, brain tumors |
AAV Vectors
AAV vectors, derived from nonpathogenic, single-stranded DNA parvoviruses, have emerged as one of the safest and most extensively used vectors for gene delivery in both basic and clinical applications due to their ability to infect nondividing cells, high transduction efficiency, long-lasting expression from a single dose, and relatively low host immune response ( ). Consequently, a majority of clinical trials addressing nononcologic diseases of the CNS have used AAV vectors ( ). More than 100 serotypes of AAV have been identified, each with a distinct tissue selectivity and transduction efficiency due to differences in its capsid proteins, and efforts to engineer AAV capsids to improve cell-type specificity and reduce immunogenicity are ongoing. The most commonly used AAV serotypes in the CNS currently are 1, 2, 4, 5, 6, 8, and 9. AAV2 has been used most extensively in clinical studies, but its spread and neuronal transduction in the brain after direct injection are limited compared with the more recently characterized AAV1, AAV5, and AAV9 serotypes ( ). Notably, AAV9 has recently been shown to cross the blood–brain barrier (BBB) after both intravenous and intra–cerebrospinal fluid (CSF) injection, inducing widespread transgene expression throughout the CNS in the absence of direct injection ( ). While AAV vectors remain at the forefront of gene therapy, their small packaging size (<4.7 kb) and slow onset of expression may limit the use of these vectors in settings where large or multiple genes may be required or very rapid expression is needed. Lastly, the recent development of a primate-derived AAV strain (rh10) for use in the human brain might ultimately mitigate ongoing concerns of immunogenicity as it may bypass the antihuman AAV immunity that is common in the population ( ).
LV Vectors
LV vectors, derived from the single-stranded RNA retrovirus HIV-1, have been used extensively as gene transfer tools in the CNS due to their ability to infect nondividing cells, efficiently integrate into the host genome, carry large transgenes, and allow for stable long-term transgene expression. LV vectors have been shown to transduce most cell types within the CNS, and clinical trials in which LV vectors were used to correct gene defects ex vivo have demonstrated their efficacy in a number of neurogenetic disorders (reviewed in ). Despite their advantages, however, LV vectors are associated with a relatively high risk of insertional mutagenesis as they integrate into the host genome, thus potentially retaining the ability to induce oncogenesis. Nonintegrating LV vectors are currently in development to overcome these concerns.
HSV Vectors
HSV vectors, derived from pathogenic double-stranded DNA viruses, have been widely used for CNS gene transfer in basic and clinical applications given their large transgene capacity, tropism for neurons, and lack of insertional mutagenesis. The vast majority of clinical trials using HSV vectors have focused on brain tumors (reviewed in ) and non-CNS cancers, but HSV vectors have also been trialed as gene therapy for pain ( ). Uniquely, HSV vectors undergo retrograde transport from the periphery to cell bodies, a property that allows long-term transgene expression in CNS neurons without direct CNS infusion, and one that has been harnessed for the ongoing development of novel therapies for chronic pain.
Adenoviral Vectors
Adenoviral vectors, derived from pathogenic double-stranded DNA viruses, have historically been considered advantageous as they transduce nondividing cells, carry large transgenes, and exhibit fast transgene expression without the risk of insertional mutagenesis. However, their applications as CNS gene therapy vectors remain limited given the transient nature of their transgene expression and their high propensity to provoke significant immune responses.
Control of Transgene Expression
While the design of all viral-based gene therapy strategies begins with selection of an appropriate vector, optimization of the viral vector genome for robust transgene expression targeted to the specific cell type or tissue of interest remains essential. As will be reviewed in depth below, many diseases of the CNS are potentially treatable with targeted gene therapy strategies, each with significant variability in pathophysiology, CNS location, affected cell-type(s), underlying genetic defect, and requirements for therapeutic gene expression or repression. Thus, the requirements for a safe and effective gene therapy vector targeting the global genetic defects characteristic of pediatric leukodystrophies will likely differ significantly from a gene therapy targeting an adult neurodegenerative disease localized to a specific brain region. However, most neurologic disorders are likely to require tightly controlled transgene expression that is limited to specific cell populations. The modification of viral vector genomes to control transgene expression or repression in multiple domains is briefly discussed here.
Promotor Selection
In addition to viral tropism for neurons, both the level of transgene expression and cell type–specific targeting can be directed by cis -acting elements contained in the vector genome, including the selection of 5′-untranslated region (UTR), 3′-UTR, enhancer, promoter, and polyadenylation signal ( ). Of these elements, the use of cell type–specific promoters greatly enhances cellular targeting as these binding sites for specific transcription factors restrict transgene expression to genetically defined cell populations. The arsenal of cell-, tissue-, and disease-specific promoters has expanded considerably in recent years, reflecting ongoing efforts to improve the strength, duration, and selectivity of transgene expression. For example, human synapsin ( hSyn ), human thymocyte antigen ( hThy1 ), and neuron specific enolase ( NSE ) are selective for neurons and glial fibrillary acidic protein ( GFAP ) for astrocytes; calmodulin kinase II alpha ( CamK11a ) selects for excitatory neurons and fugu somatostatin ( fSST ) for inhibitory neurons ( ). Although full specificity of most promoters often requires far larger DNA fragments than can be accommodated by even the largest viral vectors, improved understanding of regulatory elements and combining these into synthetic promoters should help overcome this issue and provide an expanding toolkit of regulators to improve specificity of CNS gene therapy.
Regulatable Expression Systems
The ability to control the timing of transgene expression can be achieved by using a ligand-inducible promotor, which allows pharmacologic control of transgene expression following viral vector administration in vivo. For example, many preclinical studies have used the tetracycline (Tet) on/off system whereby viral-mediated transgene expression is dependent on either the presence or absence of tetracycline and thus can be switched on or off with the oral administration of doxycycline. Other examples of ligand-inducible systems are reviewed in . Several systems have also been developed to ensure that viral-mediated transgene expression can be exquisitely restricted to genetically defined cell populations, including specific neuronal subpopulations. Cre-lox neurogenetics has emerged as one experimental platform that allows for strong transgene expression in any population of cells that coexpress the DNA recombinase Cre . In this approach, a transgene can be inserted into the viral vector genome such that it can be transcribed only after inversion by Cre in vivo (reviewed in ). The specificity of transgene expression can then come from the targeted expression of Cre in driver mouse lines in which Cre is either expressed in a specific cell type or the expression of Cre is induced by a specific external stimulus ( ), providing a means to control the precise location of transgene expression in the CNS. A related approach involves use of a tamoxifen-inducible form of Cre ( ) to regulate transgene expression. Finally, several newer strategies have been developed allowing for both temporal and spatial regulation of transgene expression and are discussed at the end of this chapter. To date, however, no strategy designed to achieve regulation of transgene expression has been successfully translated to humans, although a Phase I clinical trial for glioblastoma multiforme (GBM) using a multiple AV vector-based “Tet on” approach is being planned based on recent preclinical data ( ).
Additional Gene Therapy Approaches
Currently, gene therapy approaches are primarily focused on delivering a gene that causes a needed protein to be expressed; however, more recently, increased understanding of nuclease function has led to more direct DNA editing, using techniques such as RNA interference (RNAi) and CRISPR/Cas9 (clustered regularly interspaced short palindromic repeats/CRISPR-associated protein 9), both of which can be delivered to the CNS via viral-based methods. In the context of gene therapy, RNAi provides a mechanism for specific gene silencing via engineered short hairpin RNAs (shRNAs) and microRNAs (miRNAs), molecules that target specific gene sequences, act as posttranscriptional regulators, and can be used in conjunction with viral-mediated approaches. Briefly, shRNAs and miRNAs recognize target mRNAs and induce transcriptional repression or mRNA cleavage, depending on their partial or complete sequence complementarity, respectively ( ). The most recent addition to our toolbox for genome editing is the rapidly developing CRISPR/Cas9 system. Originally discovered in bacteria, CRISPR/Cas9 requires two molecules, a guide RNA (gRNA) complementary to a given target gene sequence and a nuclease called Cas9 ( ). The gRNA recognizes its complementary DNA sequence in the genome and Cas9, depending on the enzymatic variant, creates a single- or double-strand break in the DNA. Single-strand breaks can trigger homology directed repair (HDR), allowing for insertion of new genes into the genome and providing a mechanism for genetic correction of DNA mutations. Alternatively, double-stranded DNA breaks trigger nonhomologous end joining (NHEJ), resulting in targeted gene disruption and inactivation of the target gene. In the context of gene therapy, CRISPR/Cas9 can theoretically be used as a therapeutic tool to correct the causative gene mutations in monogenic recessive disorders or to inactivate the mutated allele in dominant-negative disorders. For example, it was recently shown that CRISPR/Cas9-mediated genome editing partially restored dystrophin expression in a mouse model of muscular dystrophy ( ).
Human Clinical Safety and Efficacy Data for CNS Gene Therapy
To date, more than 1850 gene therapy clinical trials have been completed, are ongoing, or have been approved worldwide ( ). Although overlap in gene therapy approach exists for certain CNS diseases (e.g., Parkinson disease), retroviral vectors have generally been used for ex vivo gene transfer while AAV vectors have been extensively used for in vivo gene transfer. Ex vivo refers to the genetic augmentation of cells outside the body followed by reintroduction of genetically modified cells into the patient (analogous to a bone marrow transplant), an approach that is distinct from in vivo gene transfer, where the viral vector is administered directly to the patient. Historically, ex vivo gene transfer has been used to treat the CNS in several ways: (1) hematopoietic stem cells differentiate into microglia that migrate through the brain and deliver the missing gene product to neural cells, (2) hematopoietic stem cells secrete the absent gene product into the intravascular compartment, which crosses the BBB into the brain, or (3) the immune system is corrected, profiting the nervous system. Of clinical trials focused on neurologic disorders, the vast majority have used AAV in vivo as gene therapy vector ( ). A selection of representative clinical trials of gene therapy for neurologic disease is shown in Table 30.2 .
Disease | Phase | Viral Vector | Transgene | Target/Route of Administration |
---|---|---|---|---|
Canavan Disease | ||||
I/II | AAV2 | ASPA | Intraparenchymal injection to six brain sites | |
Batten Disease | ||||
I | AAV2 | hCLN2 | Intraparenchymal injection to 12 brain sites | |
X-Linked Adrenoleukodystrophy | ||||
I | LV | ABCD1 | Ex vivo transfection of hematopoietic stem cells | |
Parkinson Disease | ||||
I | AAV2 | GAD | Stereotactic unilateral injection into subthalamic nucleus | |
II | AAV2 | GAD | Stereotactic bilateral injection into subthalamic nucleus | |
I | AAV2 | Neurturin | Stereotactic bilateral injection into putamen | |
II | AAV2 | Neurturin | ||
I | AAV2 | Neurturin | Stereotactic bilateral injection into putamen and substantia nigra | |
II | AAV2 | Neurturin | ||
I | AAV2 | AADC | Stereotactic bilateral injection into putamen | |
I | AAV2 | AADC | Stereotactic bilateral injection into putamen | |
I/II | LV | AADC/TH/GTP cyclohydrase I | Stereotactic bilateral injection into putamen | |
Alzheimer Disease | ||||
I | AAV2 | NGF | Stereotactic bilateral injection into nucleus of Meynert | |
Brain tumors | ||||
I–III | HSV1 | TK | Resection cavity | |
I–III | AV | TK | Resection cavity | |
I | AV | p53 | Intratumoral | |
I | AV | IFN-β | Intratumoral |
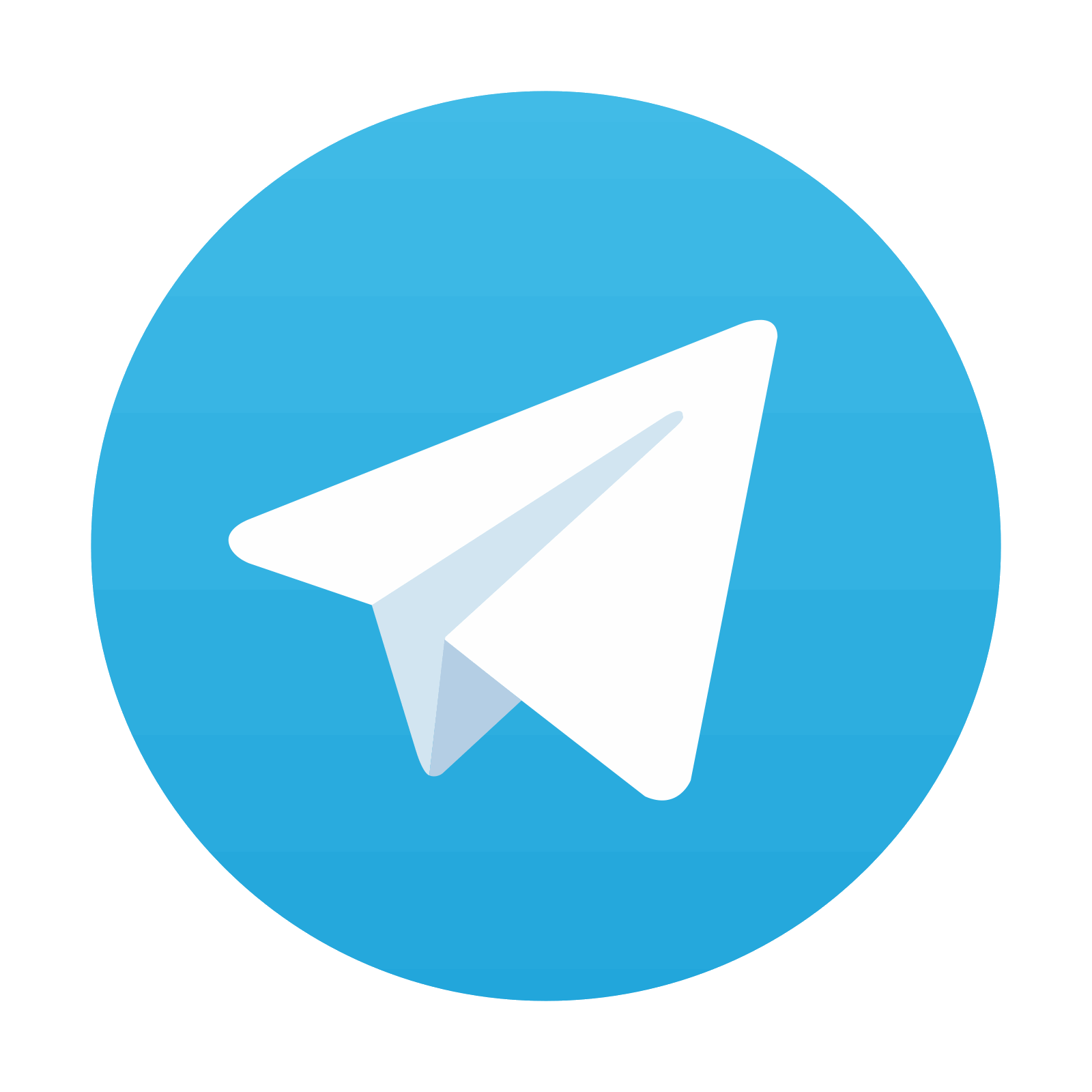
Stay updated, free articles. Join our Telegram channel
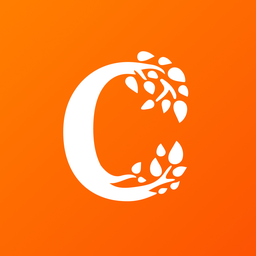
Full access? Get Clinical Tree
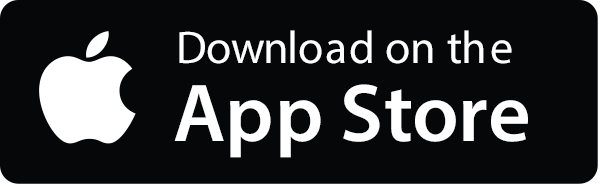
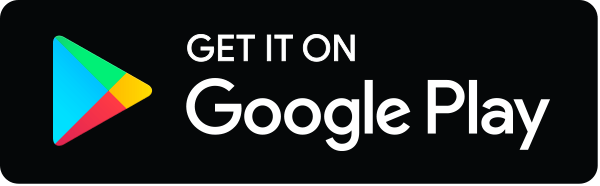
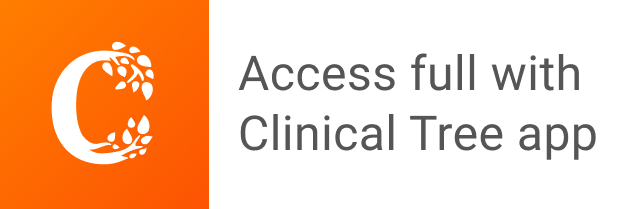