General Principles of Neurologic Localization
Introduction
Fittingly, a book on localization in clinical neurology should begin with a chapter explaining what the term localization means. Localization derives from the Latin term locus or site. Localization is the diagnostic exercise of determining from the signs (most often) or symptoms of the patient what site of the nervous system has been affected by a disease process. Important injury to the nervous system results in abnormal function, be it behavioral, motor, or sensory. Characteristics of the dysfunction often pave the way for a topographic (from the Greek term topos or place) diagnosis. Localization and topographic diagnosis refer to the same thing: the determination of where in the nervous system the damage has occurred.
Even in the age of sophisticated neurophysiology, neuroimaging, and molecular biology, the clinical diagnosis should precede the use of these other techniques if their full diagnostic potential is to be realized. Clinical localization has particular relevance to the adequate use of ancillary procedures. For instance, false-positive findings from “gunshot approach” neuroimaging can only be avoided by careful localization. As an example, congenital brain cysts, strikingly visible on imaging procedures, are often wrongly blamed for a variety of neurologic disorders, while the actual disease remains overlooked and untreated. The thoughtful use of ancillary procedures in neurology, guided by clinical localization, minimizes discomfort for patients and the waste of resources.
A Brief History of Localization: Aphasia as an Example
The history of localization is the history of early neurology, concerned with topographic diagnosis that would eventually lead to therapy. In few areas of neurology was the development of localization as interesting and so much at the center of famous controversies as it was in the case of aphasia. In fact, the oldest known document on neurologic localization concerns aphasia. It was recorded in an Egyptian papyrus from the Age of the Pyramids (about 3000–2500 BC), where an Egyptian surgeon described the behavior of an aphasic individual:
If thou examinest a man having a wound in his temple, penetrating to the bone, (and) perforating his temporal bone; … if thou ask of him concerning his malady and he speak not to thee; while copious tears fall from both his eyes, so that he thrusts his hand often to his face so that he may wipe both his eyes with the back of his hand … Edwin Smith surgical papyrus, Case 20, 2800 BC [12].
From the time of Hippocrates, in ancient Greece, it was documented that injury to the left part of the brain resulted in weakness of the right side of the body. However, paired organs in the body were thought to have identical functions. In the mid-19th century, Paul Broca (1824–1880) revolutionized the then current understanding of the functional organization of paired organs by describing lateralization of language to the left hemisphere [5,13]. He called aphemia the disorder that we now call Broca’s aphasia. In his 1865 paper, he wrote:
Now, this function of the intellectual order, which controls the dynamic element as well as the mechanical element of articulation, seems to be the nearly constant privilege of the left hemisphere convolutions, since lesions that result in aphemia are almost always localized in that hemisphere … That is tantamount to saying that we are left-brained with regard to language. Just as we control movements in writing, drawing, embroidering, etc, with the left hemisphere, so we speak with the left hemisphere.
Broca defined the inferior frontal gyrus as the area that, when injured, would lead to aphemia [13]. He also noted the variation in the expression of diverse lesions in the inferior frontal gyrus, characteristic of the plasticity found in cortical organization:
During the course of our study of brains of patients with aphemia, many times before, we had determined that the lesion of the third left frontal convolution was not always in direct relation to the intensity and the impairment of language. For example, we had observed that speech was completely wiped out as a result of a lesion with the size of 8 to 10 mm, whereas, in other cases, lesions that were tenfold more extensive had only partly impaired the capacity for articulate speech.
In the few years after Broca’s remarkable statements, knowledge about the localization of the language centers in the brain grew rapidly. Already in 1874, Carl Wernicke (1848–1905) wrote:
The whole area of convolution encircling the Sylvian fissure, in association with the cortex of the insula, serves as a speech center. The first frontal gyrus, being motor, is the center for representation of movement, and the first temporal gyrus, being sensory, is the center for word images … The first temporal gyrus should be considered as the central end of the auditory nerve, and the first frontal gyrus (including Broca’s area) as the central end of the nerves to the speech muscles … Aphasia can result from any interruption of this path …
Knowledge of the cortical organization for language had been derived from careful clinicopathologic correlation [67]. After describing a 73-year-old woman with the sudden onset of confused speech, Wernicke goes on to describe the pathologic findings:
The branch of the artery of the left Sylvian fissure, running down into the inferior sulcus of Burdach, was occluded by a thrombus tightly adherent to the wall. The entire first temporal gyrus, including its junction with the second temporal gyrus and the origin of the latter from Bischof’s inferior parietal lobule were converted into a yellowish-white brei [67,68].
Wernicke’s diagram of the language areas is illustrated in Figure 1.1.
Current techniques, such as functional brain mapping, promise to clarify further the localization of mechanisms underlying neurologic dysfunction. For instance, conduction aphasia, initially described by Wernicke in 1874, has traditionally been associated with damage of the arcuate fasciculus, purportedly connecting Wernicke’s with Broca’s area. Recent neurophysiological and neuroimaging findings, obtained with the use of diffusion tensor imaging and other functional magnetic resonance imaging (MRI) techniques, are challenging this notion [6].
Clinical Diagnosis and Lesion Localization
Clinical diagnosis in neurology requires several steps:
1. Recognition of impaired function
2. Identification of what site of the nervous system has been affected, that is, localization
3. Definition of the most likely etiology, often resulting in a differential diagnostic list
4. Use of ancillary procedures to determine which of the different possible etiologies is present in the given patient
FIG. 1.1. Wernicke’s diagram of the language areas. In the original, the label on the superior temporal gyrus was simply a, but from the context, it should have been a1. Wernicke’s explanation of this figure is as follows:
Let F be the frontal, O the occipital, and T the temporal end of a schematically drawn brain. C is the central fissure; around the Sylvian fissure (S) extends the first primitive convolution. Within this convolution, ai is the central end of the acoustic nerve, a its site of entry into the medulla oblongata; b designates the representation of movements governing sound production, and is connected with the preceding through the association fibers ai b running in the cortex of the insula. From b the efferent pathways of the sound-producing motor nerves run to the oblongata and exit there …
(From: Wernicke C, Der aphasische symptomencomplex; eine psychologische studie auf anatomischer basis, Breslau: Max Cohn & Weigert, 1874 [67].)
Each of these steps is important. The first one, recognition of impaired function, depends on a good history and neurologic examination. Only by storing the range of normal neurologic functions in their mind can physicians recognize an abnormal neurologic function. Inexperience or carelessness in examining a patient often results in overlooking a neurologic deficit and therefore missing a diagnosis. For instance, mild chorea may appear to the inexperienced as normal fidgetiness. The slow eye movements of a pontocerebellar disorder may pass completely unrecognized by someone who looks only for a full excursion of the eyes.
Abnormal neurologic findings come in the form of abnormal behavior, impaired posture or gait, difficulty with movements of the face or extremities, and, finally, sensory disturbances, including pain. Pain exemplifies well several of the difficulties physicians face when confronting possible neurologic dysfunction.
1. First, is the dysfunction real? Is the pain really there or is the patient trying to deceive? We have witnessed the plight of a paraplegic patient who had been repeatedly asked by health care personnel to stop pretending not to be able to move his legs. They had misinterpreted the triple flexion response witnessed when they pulled the sheets off the patient’s legs as evidence of volitional movement. Movement disorders, such as the dystonias, were frequently considered psychogenic in the past and have gradually emerged from this realm into a phase of general recognition of their “organicity.” Unless accompanied by clear psychiatric manifestations, neurologic symptoms or signs should be taken at face value.
2. Second, to what extent is the dysfunction pathologic, that is, indicative of injury serious enough to warrant a formal diagnostic workup? Many aches and pains do not reflect serious disease. Sending everyone with a “little pain” to a physician would hopelessly clog up the health care system. Interestingly, the child learns from falls and other minor injuries what to expect as “normal pain,” and when a person seeks medical attention for any symptom, the likelihood is that the problem is serious enough to warrant at least a thoughtful physical examination.
3. Third, is the dysfunction neurologic in origin? Is the pain due to injury of the affected body part or neurologic dysfunction? Is the dysfunction a manifestation of a disease of the nervous system rather than of the organ mediating the function? Is the patient unable to walk because of arthritis or because the motor system is affected? All these questions find an answer when the physician recognizes patterns that belie neurologic impairment, for instance, in the case of pain, a characteristic radicular nature and distribution. In other cases, the neurologic examination may reveal other manifestations of unquestionable neurologic dysfunction. A patient with pain in the hand may also have atrophy of the muscles in the thenar eminence and a Tinel’s sign—pain on percussion of the median nerve at the wrist. Knowledge of localization tells us that the pain derives from injury of the median nerve at the point where the pain increases on percussion. What is needed to localize the lesion, in this case as in any other, is a good working knowledge of neuroanatomy.
Neuroanatomy is a key to localization. In this book, a synopsis of the anatomy of each structure of the nervous system precedes the discussion on localization of lesions of that structure. Neuroanatomy has two broad aspects: the morphology of the structure and its “functional representation.” Functional representation refers to the function mediated by a given structure of the nervous system. Damage to the structure alters the function mediated by this structure. For example, an injury to the oculomotor nerve results in mydriasis in the eye supplied by this nerve.
Neuroanatomy provides the road map for localization. Localizing is identifying the site of injury on the neuroanatomic map. As with any other map, we need either an address, with street name and number, or the intersection between two well-defined streets or roads. Injury expresses itself through neurologic dysfunction, be it behavioral, motor, or sensory. If we know what kind of dysfunction can result from injury of the different parts of the nervous system, we will be able to identify the source of the injury. Some types of dysfunction directly give us the address we are looking for. A combination of resting tremor, bradykinesia, and rigidity tells us that the substantia nigra of the patient has been injured. At other times, we use the approach of looking for the intersection between two streets. From some signs we deduce that a particular pathway must be affected. From others, we infer that a second pathway is affected as well. The injury must be in the place where these pathways meet. For instance, by the presence of left-sided hemiparesis we infer that the corticospinal tract has been affected. But the corticospinal tract can be affected at the level of the spinal cord, brainstem, or cerebral hemispheres. To precisely identify the location of damage we need to use other clues. If, in addition to the left-sided hemiparesis, we find a right third nerve palsy, we are well on our way to localizing the lesion. This well-known syndrome, named after Weber, typifies a general principle of localization: the lesion is where the two affected pathways cross. If the patient only had a third nerve palsy, the lesion could be anywhere between the fascicle of the nerve (in the brainstem) and the superior orbital foramen (in the orbit). The addition of a contralateral hemiparesis precisely defines that the lesion affects the crus cerebri on the same side of the third nerve palsy. This is where the corticospinal tract and the fibers of the third nerve meet. Neuroanatomy provides the roadmap for a correct assessment.
Localization tends to be more precise when the lesion affects the lower levels of the nervous system. When we localize lesions of the nervous system, it is helpful to think about the major syndromes that result from lesions at different functional and anatomic levels, from the muscle to the cortex. At the simplest level, injury to a muscle impairs the movement mediated by that muscle. One level higher, we find that injury to a peripheral nerve causes weakness of the muscles innervated by that nerve and sensory loss in its cutaneous distribution. Lesions in the spinal cord below the low cervical level cause weakness of one or both legs and sensory loss that often has a horizontal level in the trunk. Lesions in the cervical cord or brainstem typically cause weakness or sensory loss on one or both sides of the body, often more severe on one side, and findings characteristic of the level affected. For instance, lesions of the cervical cord may cause radicular pain or weakness affecting the arms or hands. Lesions of the lower pons give rise to gaze palsies or peripheral facial weakness. The localization of lesions in the cranial nerves (CNs) is fairly straightforward because they may affect a peripheral nerve or a neuroanatomic structure that is relatively simple, such as the visual pathways. As we ascend the neuraxis, the localization of lesions becomes less precise. Lesions in the cerebellum may cause ataxia. Lesions in the thalamus often, but not always, cause sensory loss and postural disorders, or memory loss. Lesions in the hemispheric white matter may give rise to weakness or visual field defects. Finally, lesions in the cortex manifest themselves by an array of motor, sensory, or behavioral findings that vary according to the area that has been injured.
Similarly, lesions of the lower levels tend to cause findings that change little over time, whereas lesions of the higher levels may be very “inconsistent” in the course of an examination. An ulnar nerve lesion may be responsible for atrophy of the first dorsal interosseous muscle. The atrophy diagnosed by the examiner will be consistent. By contrast, a patient with a Broca’s aphasia may have a great deal of difficulty repeating some words, but not others of apparently similar difficulty. The examiner may be puzzled and not know what to document: can the patient repeat or can she not? In this case, what should be noted is not whether the patient can do something, but whether she does it consistently in a normal way. Any difficulty repeating a sentence on the part of a native speaker of a language should be considered as abnormal. Higher neurologic function should be sampled enough to avoid missing a deficit that the more complex neural networks of higher levels can easily mask.
For the anatomic localization of lesions, the neurologic examination is much more important than the history. It must be noted that when we speak here about “examination,” we include the sensory findings reported by the patient during the examination. A complaint of pain or of numbness is usually as “objective” as a wrist drop. By tracking back the pathways that mediate the functions that we find are impaired in the neurologic examination, we can generally localize the site of the lesion, even without a history. The history, that is, the temporal evolution of the deficits witnessed in the neurologic examination, is important in defining the precise etiology. For instance, a left-sided hemiparesis is detected in the neurologic examination. If it occurred in a matter of minutes, cerebrovascular disease or epilepsy is most likely. If it evolved over a few days, we should think about an infection or demyelinating disease. If it developed insidiously, in a matter of months, a tumor or a degenerative process is more likely. In all of these cases, the localization is derived from the findings of the examination: we detect a left-sided hemiparesis. If we also find a right third nerve palsy and determine that it has appeared at the same time as the hemiparesis, we will emphasize the need for a careful look at the midbrain when we obtain an MRI. In this sense, the history is also important for localization: we may witness in the examination the end result of multiple lesions that affected the nervous system over time. In the previous example, if the third nerve palsy occurred when the patient was 10 years old and the hemiparesis appeared when he was in his sixties, the lesion responsible for the hemiparesis would probably not be in the midbrain.
Finally, there is the issue of discrete lesions versus system lesions. Much of the work on localization has been done on the basis of discrete lesions, such as an infarct affecting all the structures in the right side of the pons. Some types of pathologies tend to cause this type of lesion. Cerebrovascular disease is the most common, but demyelinating lesions, infections, trauma, and tumors also often behave like discrete, single, or multiple lesions. Other neurologic disorders affect arrays of neurons, often responsible for a functional system. Parkinson’s disease is an example. Here, the localization to the substantia nigra is simple. The localization of other degenerative disorders, such as the spinocerebellar degeneration of abetalipoproteinemia or vitamin E deficiency, is more complicated [56]. Here, the clinical syndrome seems to point to the spinal cord, but the real damage is inflicted to the large neurons in the sensory nuclei of the medulla, dorsal root ganglia, and Betz cells. The puzzle is resolved when one realizes that the destruction of the corticospinal tract logically follows metabolic injury to the neurons that give rise to it. The larger neurons, with the longest axons reaching the lumbar segments, are affected first. The neuron may not die, but, incapable of keeping an active metabolism, it begins to retract its axon (dying-back phenomenon). Likewise, the lesion in the dorsal columns of the spinal cord (and peripheral nerve) simply reflects the damage inflicted to the larger sensory neurons by the lack of vitamin E. Therefore, a precise knowledge of the functional significance of the different structures of the neuraxis facilitates the localization of degenerative or system lesions as much as it helps with discrete lesions.
Having reviewed some general principles of localization in the nervous system, we will now review in more detail the principles of localization in the motor and sensory systems. Finally, we will review the localization of gait disorders.
Localization of Lesions of the Motor System
Anatomy of the Motor System
The motor neurons of the ventral horn of the spinal cord and the motor nuclei in the brainstem, whose axons synapse directly on striated muscles, are the “final common pathway” for muscle control. These large alpha (a) motor neurons supply the extrafusal fibers of the skeletal muscles providing the only axons to skeletal muscle. Scattered among the a motor neurons are many small gamma (g) motor neurons, which supply the intrafusal fibers of the muscle spindles. These muscle spindles are the receptors for the muscle stretch reflexes. The motor neuron, together with its axon, and all the muscle fibers it supplies, is called the motor unit. The junction between the terminal branches of the axon and the muscle fiber is called the neuromuscular junction [23,49]. There is a somatotopic organization of the cell columns of anterior horn cells in the ventral gray horn of the spinal cord. Neurons that supply the axial muscles, including the neck muscles, are located in ventromedially placed columns; neurons that supply proximal muscles are situated in the midregion; and neurons supplying the musculature of the distal aspect of the limbs are located in laterally placed columns [23,49].
Motor neuronal cell groups receive input from the contralateral motor cortex (MC) through the descending corticospinal and corticobulbar tracts (Figs. 1.2A and B). The corticospinal tract contains on each side approximately 1 million fibers of various sizes, but only 3% of all the fibers originate from the giant pyramidal cells of Betz found in layer V in the primary MC. All corticospinal fibers are excitatory and appear to use glutamate as their neurotransmitter. The neurons from which the corticospinal and corticobulbar tracts arise are known as upper motor neurons [2,4,23,31,66]. The corticospinal pathway, which controls voluntary, discrete, highly skilled movements of the distal portion of the limbs, arises from somatotopically organized areas of the primary MC, lateral premotor cortex (PMC), and supplementary motor area (SMA). These fibers arise from both precentral (60%) and postcentral (40%) cortical areas. The corticospinal neurons are found primarily in Brodmann’s area 4 (40%), which occupies the posterior portion of the precentral gyrus (primary MC). The lateral PMC, on the lateral aspect of the frontal lobe, and the SMA, on its medial part, are located in Brodmann’s area 6 (20%). Corticospinal axons also arise from neurons in the primary sensory cortex in the postcentral gyrus (Brodmann’s areas 3, 1, and 2), particularly from area 3a, anterior paracentral gyri; superior parietal lobule (Brodmann’s areas 5 and 7); and portions of the cingulate gyrus on the medial surface of the hemisphere. Fibers of the corticospinal system descend in the corona radiata, the posterior limb of the internal capsule, the middle three-fifths of the cerebral peduncle, the basis pontis (where the tract is broken into many bundles by the transverse pontocerebellar fibers), and the medullary pyramids.
In the caudal end of the medulla, nearly 75% to 90% of the corticospinal fibers in the pyramid cross the ventral midline (pyramidal decussation or Mistichelli crossing) before gathering on the opposite side of the spinal cord as the lateral corticospinal tract. In the posterior limb of the internal capsule, the corticospinal tract is organized somatotopically, with hand fibers lateral and slightly anterior to foot fibers [32]. The corticospinal fibers also follow a somatotopic organization in the pons. Fibers controlling the proximal muscles are placed more dorsal than those controlling the more distal muscle groups. Because of the ventral location of the pyramidal tract in the pons, a pure motor hemiparesis of brainstem origin is usually observed with pontine lesions. Unilateral motor deficits may predominantly involve the upper or lower limb, but a difference in the pontine lesion location among these patterns of weakness distribution is not observed [43]. There is also a somatotopic organization of the corticospinal fibers within the medullary pyramids, with fibers of the lower extremities placed more laterally and decussating more rostrally than those of the upper extremities [24]. The remaining fibers that do not decussate in the medulla descend in the ipsilateral ventral funiculus as the ventral or anterior corticospinal tract (Türck’s bundle). Most of these fibers ultimately decussate at lower spinal cord levels as they further descend in the anterior column of the spinal cord. Therefore, only approximately 2% of the descending corticospinal fibers remain truly ipsilateral, forming the bundle of Barnes [2]. These ipsilateral descending projections control the axial musculature of the trunk and proximal limbs.
FIG. 1.2. A simplified diagram of the motor system. A: Corticospinal tract. B: Corticobulbar tract.
The corticobulbar fibers, originating in the lower third of the cortical motor fields, especially the MC and SMA, descend in the genu of the internal capsule, the medial part of the cerebral peduncle, and the basis pontis, where they are intermixed with corticospinal fibers. The corticobulbar pathway has bilateral input to the nuclei of the trigeminal and hypoglossal CNs, as well as the facial nucleus supplying the upper facial muscles. Traditional localization concepts postulate that ventral brainstem lesions rostral to the lower pons result in contralateral central facial paresis, whereas lesions of the lower dorsolateral pons result in ipsilateral facial paresis of the peripheral type. However, an aberrant fiber bundle branching off the main pyramidal tract at the midbrain and upper pons, along the tegmentum in a paralemniscal position, has been described. Therefore, whereas the muscles of the lower face receive predominantly crossed corticobulbar input, the muscles of the upper face are represented in the ipsilateral, as well as the contralateral, hemisphere, with transcranial magnetic stimulation (TMS) studies showing that the amount of uncrossed pyramidal projections are no different from the muscles of the upper than those of the lower face [21,61]. TMS studies in patients with and without central facial paresis due to brainstem lesions have also shown that a supranuclear facial paresis may be contralateral to a lesion of the cerebral peduncle, pontine base, aberrant bundle or ventral medulla, or ipsilateral to a lateral medullary lesion [64].
The ventral part of the facial nucleus, innervating the lower two-thirds of the face, has a predominantly crossed supranuclear control. This schema of supranuclear facial muscle control holds true for voluntary facial movements. Emotional involuntary movements and voluntary facial movements may be clinically dissociated, and therefore, a separate supranuclear pathway for the control of involuntary movements probably exists. A prevailing view is that the SMA and/or cingulate motor areas are critical for emotional facial innervation [35]. Fibers mediating emotional facial movements do not descend in the internal capsule in their course to the facial motor nuclei. The right cerebral hemisphere is also involved in supranuclear emotional facial movement control and is “dominant” for the expression of facial emotion [10]. Furthermore, some of the facial corticobulbar fibers seem to descend ipsilaterally before making a loop as low as the medulla and decussating and ascending to the contralateral facial nucleus (located dorsolaterally in the caudal pons) that innervates the perioral facial musculature [17,62]. This anatomic understanding explains the emotional facial paresis of pontine origin resulting from the involvement of the dorsal lateral pontine area [33].
Within the MC, corticospinal neurons are somatotopically organized in patterns that reflect their functional importance (motor homunculus). The size of the cortical representation in the motor homunculus varies with the functional importance of the part represented; therefore, the lips, jaw, thumb, and index finger have a large representation, whereas the forehead, trunk, and proximal portions of the limbs have a small one. As an example, isolated hand weakness of cortical origin may present with loss of thumb and finger movements and impaired hand flexion and extension or with partial involvement of a few digits (pseudoradicular pattern). This cortical motor hand area has been localized in the middle to lower portion of the anterior wall of the central sulcus (Brodmann’s area 4), adjacent to the primary sensory cortex of the hand (Brodmann’s areas 3a and 3b) [60]. Neurons in the medial aspect of the MC and the anterior paracentral gyrus influence motor neurons innervating the muscles of the foot, leg, and thigh. Neurons in the medial two-thirds of the precentral gyrus influence motor neurons innervating the upper extremity and trunk. Neurons in the ventrolateral part of the precentral gyrus contribute to the corticobulbar tract and project to motor nuclei of the trigeminal (CN V), facial (CN VII), glossopharyngeal (CN IX), vagus (CN X), accessory (CN XI), and hypoglossal (CN XII) nerves to influence the cranio–facial–oral musculature [4,31]. As an example, each hypoglossal nucleus receives impulses from both sides of the cerebral cortex, except for the genioglossus muscle that has probably crossed unilateral innervation. Therefore, a lingual paresis may occur with lesions at different anatomic levels including the medulla, hypoglossal foramen, cervical (neck) region, anterior operculum, and posterior limb of the internal capsule [26].
Sensory cortical pathways (e.g., thalamocortical connections), corticofugal projections to reticulospinal and vestibulospinal tracts, direct corticospinal projections to the spinal cord, and projections to the basal ganglia and cerebellum have an active role in the planning and execution of movements. The cerebellum and basal ganglia are critically important for motor function [2,4,66]. The cerebellum has a major role in the coordination of movements and control of equilibrium and muscle tone. The cerebellum controls the ipsilateral limbs through connections with the spinal cord, brainstem, and contralateral MC through the thalamus. A corticofugal pathway of major clinical importance is the corticopontine pathway, which arises primarily from the precentral and postcentral gyri, with substantial contributions from the PMC, SMA, and posterior parietal cortices, and few from the prefrontal and temporal cortices. These fibers descend in the anterior limb of the internal capsule and the medial fifth of the cerebral peduncle before reaching the basis pontis, where they project to pontine nuclei. Second-order neurons from pontine nuclei cross to the contralateral basis pontis and give rise to the pontocerebellar pathway.
The basal ganglia play a major role in the control of posture and movement and participate in motor planning through reciprocal connections with ipsilateral MC. The corticostriate pathway includes direct and indirect projections from the cerebral cortex to the striatum. Corticostriate projections arise mainly from motor–sensory cortex (Brodmann’s areas 4 and 3, 1, and 2), PMC (Brodmann’s area 6), and frontal eye fields (Brodmann’s area 8). Direct corticostriate projections reach the striatum through the internal and external capsules and the subcallosal fasciculus. The indirect pathways include the cortico–thalamo–striate pathway, collaterals of the corticoolivary pathway, and collaterals of the corticopontine pathway. All parts of the cerebral cortex give rise to efferent fibers to the caudate and putamen. Cortical association areas project mainly to the caudate nucleus, whereas sensorimotor areas project preferentially to the putamen. These corticostriate projections mainly terminate ipsilaterally in a topographic pattern (e.g., the frontal cortex projects fibers to the ventral head of the caudate and rostral putamen). The cortex also sends fibers to the substantia nigra, subthalamic nucleus, and claustrum.
Another corticofugal tract of major clinical importance is the corticothalamic pathway. This pathway arises from cortical areas receiving thalamic projections and, therefore, serves as a feedback mechanism from the cortex to the thalamic nuclei. Except for the reticular nucleus of the thalamus, examples of such reciprocal connections include the anterior nucleus and the posterior cingulate cortex, the ventral lateral nucleus and the MC, the ventral anterior nucleus and the SMA, the ventral posterior nucleus and the primary sensory cortex, the lateral geniculate body and the primary visual cortex, the medial geniculate body and the primary auditory cortex, and the dorsomedial nucleus and the prefrontal cortex. Corticothalamic fibers descend in various parts of the internal capsule and enter the thalamus in a bundle known as the thalamic radiation.
Additional corticofugal tracts include the corticoreticular pathway, which arises from one cerebral hemisphere, descends in the genu of the internal capsule, and projects to both sides of the brainstem reticular formation, and the highly integrated corticohypothalamic tract, which arises from the prefrontal cortex, cingulate gyrus, amygdala, olfactory cortex, hippocampus, and septal area. Corticofugal areas from the frontal eye fields (Brodmann’s area 8) and the middle frontal gyrus (Brodmann’s area 46) project to the superior colliculus and centers in the brainstem reticular formation that influence the motor nuclei of the oculomotor (CN III), trochlear (CN IV), and abducens (CN VI) nerves [7].
The internal capsule, a compact lamina of white matter, contains afferent and efferent nerve fibers passing to and from the brainstem to the cerebral hemispheres, that is, continuous rostrally with the corona radiata and caudally with the cerebral peduncles. Located medially between the caudate nucleus and the thalamus, and laterally in the lenticular nucleus (globus pallidus and putamen), in a horizontal (Flechsig) section, the internal capsule is somewhat curved with its convexity inward. The prominence of the curve (genu) projects between the caudate nucleus and the thalamus. The portion in front of the genu is called the anterior limb, which measures approximately 2 cm in length and separates the lenticular nucleus from the caudate nucleus (lenticulocaudate segment of the internal capsule). The portion behind the genu is the posterior limb, which measures 3 to 4 cm in length and separates the lenticular nucleus from the thalamus (lenticulothalamic segment). The internal capsule extends further to include sublenticular and retrolenticular segments.
The anterior limb of the internal capsule contains frontopontine fibers, and thalamocortical and corticothalamic fibers (reciprocally connecting the frontal lobe to the thalamus), as well as caudatoputaminal fibers. Corticobulbar fibers, and perhaps motor corticopontine fibers, occupy the genu of the internal capsule. This fiber arrangement explains the facial and lingual hemiparesis with mild limb involvement observed in the capsular genu syndrome [9]. In the caudal half of the posterior limb of the internal capsule, the corticospinal bundle is somatotopically organized in such a way that the fibers to the upper extremity are located more anteriorly (i.e., shoulder, elbow, wrist, and fingers), followed by fibers to the trunk and then by the fibers to the lower extremity (i.e., hip, knee, ankle, toes), bladder, and rectum. As the corticospinal tract descends through the internal capsule, its fibers intermix with other fiber systems including corticorubral, corticoreticular, and corticopontine fibers. Corticorubral, corticothalamic, and thalamocortical fibers (carrying sensory tracts from the thalamus to the parietal lobes) are also located dorsal to the corticospinal fibers, in the posterior limb of the internal capsule. Finally, the sublenticular segment of the internal capsule contains the auditory and visual radiations, while the retrolenticular segment contains the visual radiations of Gratiolet’s radiating fibers and corticotectal, corticonigral, and corticotegmental fibers. The anterior limb of the internal capsule receives its vascular supply from the artery of Heubner, a branch of the anterior cerebral artery; the genu and the middle and inferior part of the posterior limb receive their blood supply from the anterior choroidal artery; while the superior aspect of the anterior and posterior limb of the internal capsule receives their blood supply from the lenticulostriates, branches of the middle cerebral artery.
TABLE 1.1 Medical Research Council’s Scale for Assessment of Muscle Power
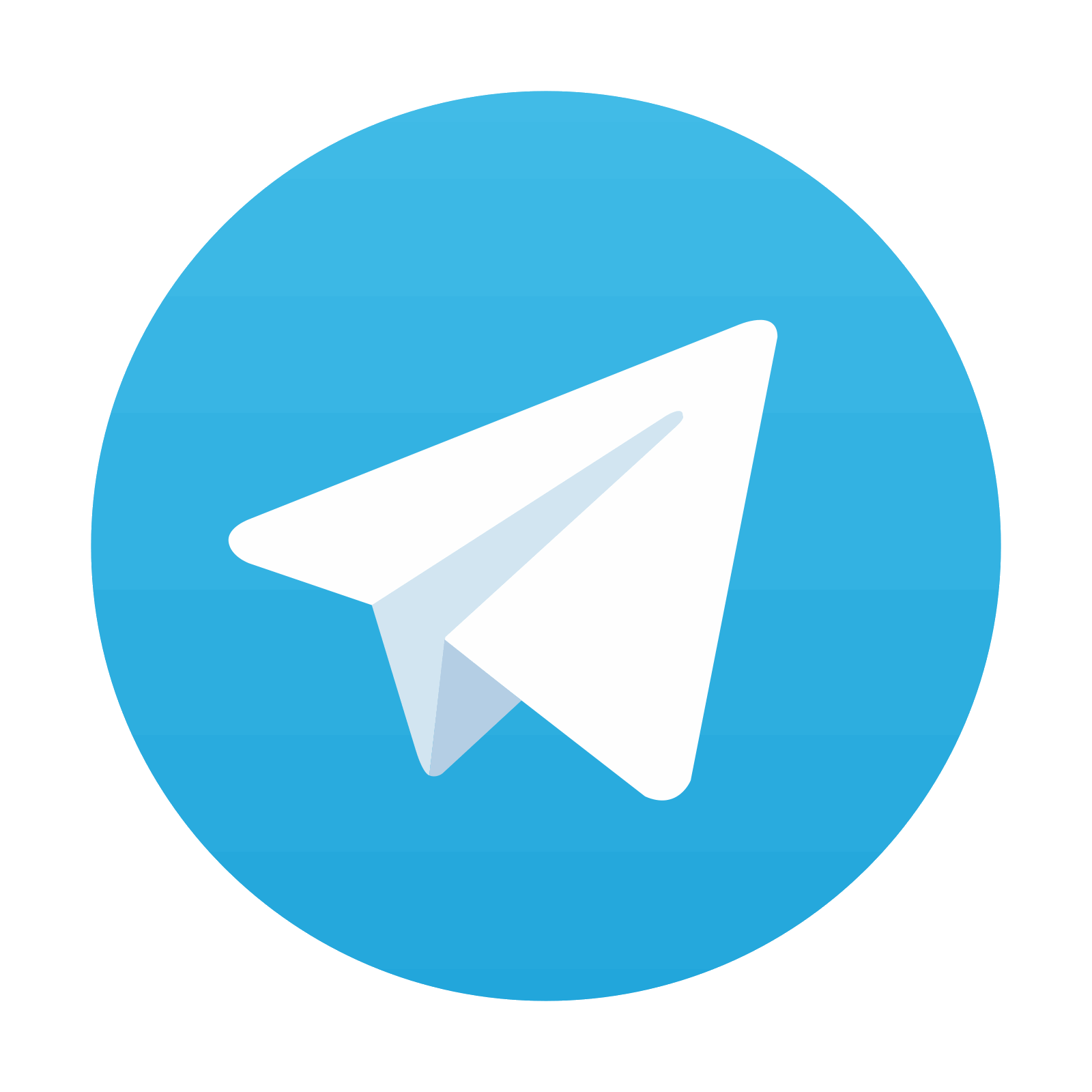