Glutamate Metabolism
Abstract
The majority of energy consumed by the brain is used to maintain membrane potentials of neurons after action potentials are fired. Glutamate, the main neurotransmitter in the central nervous system (CNS), is responsible for a large percentage of this energy expenditure. Accumulation of glutamate, however, can be toxic to neurons and, therefore, must be tightly regulated. The brain uses a combination of the blood–brain barrier, powerful glial transporters, metabolic enzymes, and glutamate cycling to maintain low extracellular levels of glutamate. These protective mechanisms, however, can become dysregulated in epilepsy. Both human and animal studies have shown that glutamate uptake into astrocytes is hindered, the metabolic processing of glutamate is slowed, and the cycling of glutamate to glutamine is downregulated in epileptic tissue. Future studies should be aimed at furthering our understanding of the functional consequences of these changes.
Keywords
Excitatory amino acid transporter (EAAT); GLT1; GLAST; EAAC1; glutamine synthetase (GS); astrocyte–neuron lactate shuttle; (ANLS); glutamate metabolism
Overview
The majority of energy consumed by the brain is used to maintain membrane potentials of neurons after action potentials are fired. Glutamate, the main neurotransmitter in the central nervous system (CNS), is responsible for a large percentage of this energy expenditure. Accumulation of glutamate, however, can be toxic to neurons and, therefore, must be tightly regulated. The brain uses a combination of the blood–brain barrier (BBB), powerful glial transporters, metabolic enzymes, and glutamate cycling to maintain low extracellular levels of glutamate. These protective mechanisms, however, can become dysregulated in epilepsy. Processes involved in glutamate homeostasis and the implications of an impaired regulatory system in epilepsy will be discussed in detail. Both human and animal studies have shown that glutamate uptake into astrocytes is hindered, the metabolic processing of glutamate is slowed, and the cycling of glutamate to glutamine is downregulated in epileptic tissue.
Metabolism in the Brain
Under basal conditions, glucose, a six-carbon sugar, is the primary metabolic substrate for the brain. Glucose is almost entirely oxidized to carbon dioxide and water, although other fates for glucose are possible. Along with O2, it is supplied via the blood circulation. The brain has a high energy demand; despite representing only about 2% of total body mass, the brain requires about 20% of the oxygen and 25% of the glucose consumed by the human body [1]. Most of the energy consumed is used to maintain the membrane potential of neurons. Glucose can enter cells through glucose transporters (GLUTs) and it is immediately phosphorylated by hexokinase to produce glucose-6-phosphate (G6P). From there, G6P can be metabolized in a number of different ways: (1) It can continue through glycolysis, ultimately committing to the breakdown of G6P to produce ATP; (2) G6P may enter the pentose phosphate pathway to ultimately generate reducing equivalents in the form of NADPH; or (3) It may be stored as glycogen (only in astrocytes). In addition, glucose can be used to synthesize lipids, amino acids, or neurotransmitters, if necessary.
Glycolysis is the metabolic pathway that converts glucose into pyruvate, a three-carbon α-keto acid. The fate of pyruvate will depend on the ratio of reducing equivalents in the brain, namely the ratio of NADH to NAD+ available in the cell. When the NADH:NAD+ ratio is low, pyruvate is preferentially converted to acetyl coenzyme A (acetyl-CoA), enters the tricarbocylic acid (TCA) cycle and is oxidized to produce energy and reducing equivalents of NADH. When the ratio of NADH:NAD+ is high, however, pyruvate may be converted to lactate via the enzyme lactate dehydrogenase (LDH). Subsequently, NADH is oxidized to NAD+ in that same process. Over the last couple of decades, lactate has received a great deal of attention as an alternative metabolic substrate in the brain.
Astrocyte–Neuron Lactate Shuttle Model
It is widely accepted that glucose is the primary energy source for the brain and, under basal physiological conditions, glucose is almost entirely oxidized to provide the necessary energy to support brain function. Other sources of energy such as ketones, fatty acids, acetate, lactate, and certain amino acids, however, may be used when supplemental energy is needed. A popular alternative energy source to glucose is lactate. Several studies have provided evidence that under certain circumstances, lactate may be preferentially metabolized by neurons [2–8]. Initially proposed in 1994 by Pellerin and Magistretti [3], this idea became known as the astrocyte–neuron lactate shuttle (ANLS) model [4]. In this model, glutamate stimulates glycolysis and the subsequent release of lactate from astrocytes, which can then be taken up by neurons and metabolized via the tricarboxylic acid (TCA) cycle. Growing evidence over the years has helped expand the model to include glutamate-stimulated aerobic glycolysis and glycogenolysis (Figs. 9.1 and 9.2) [2].

Glucose, the main cerebral energy substrate in the adult brain under normal conditions, and O2 are supplied via the blood circulation to parenchymal cells. In astrocytes, glucose is taken up via the glucose transporter GLUT1. Part of it is processed oxidatively via the TCA cycle in mitochondria while the remaining part is converted to lactate, which is released via MCT1 and MCT4 in the extracellular space. In neurons, glucose is transported via the glucose transporter GLUT3. In parallel, lactate from the extracellular space is taken up via the monocarboxylate transporter MCT2. Pyruvate arising from both glucose and lactate is used oxidatively in mitochondria to satisfy neuronal resting energy needs. It cannot be ruled out that neurons could produce a certain amount of lactate but it is considered that they have a net lactate consumption. Overall, glucose is almost entirely oxidized to CO2 and H2O within the brain, yielding a net O2/glucose consumption ratio close to six. Source: Recreated with permission from Pellerin L, Bouzier-Sore AK, Aubert A, Serres S, Merle M, Costalat R, et al. Activity-dependent regulation of energy metabolism by astrocytes: an update. Glia 2007;55(12):1251–1262.

(A) Early phase. Activation of glutamatergic afferents leads to synaptic release of glutamate, AMPA receptor (AMPAR) activation and generation of an excitatory postsynaptic potential (EPSP) caused by Na+ entry within the postsynaptic spine (1). Depolarization propagates to the dendrite and causes opening of voltage-sensitive Na+ channels, leading to further Na+ entry. Reestablishment of ion gradients is accomplished by the Na+/K+-ATPase (2) that creates considerable energy expenditure. As a consequence, oxidative phosphorylation is activated (3) and mitochondrial NADH levels first decrease [9]. Then, enhanced TCA cycle activity will ensure (4) a supply of NADH for oxidative phosphorylation and support ATP production. As pyruvate utilization in the TCA cycle increases and its cytoplasmic levels decrease, the conditions become favorable for both enhanced glucose and lactate use. Surprisingly, activation of AMPARs and coupled Na+ entry lead to a reduction in glucose uptake and utilization in neurons (5) [10], thus further favoring lactate utilization as preferential oxidative substrate (6) [11]. This would cause a transient drop in extracellular lactate levels as measured in vivo [12,13]. (B) Late phase. Glutamate released in the synaptic cleft is taken up by astrocytes to be recycled via the specific glutamate transporters GLAST and GLT1 (1). A large Na+ influx caused by glutamate uptake takes place and activates the Na+/K+-ATPase (2) [14], glucose transport (3) [15], and (4) glucose utilization [3] in astrocytes. The enhancement of aerobic glycolysis in astrocytes first causes a large increase in cytosolic NADH [9] that normalizes with the conversion of pyruvate into lactate and its release via monocarboxylate transporters expressed on astrocytes (mainly MCT1 and MCT4) (5). Such a lactate release following glutamatergic activation corresponds to the increase in extracellular lactate levels measured in vivo [12,16]. Lactate produced by astrocytes during this later phase of activation not only replenishes the extracellular pool but also could help sustain neuronal energy needs as activation persists. Metabolic events occurring in the early and late phases described earlier constitute the so-called astrocyte–neuron lactate shuttle (ANLS) and its importance grows with the degree of glutamatergic activation. Such a view is supported by a series of experiments conducted in vivo [5–7]. (C) Intense and prolonged stimulation. Upon strong and long-lasting stimulation that occurs in certain conditions, glucose utilization becomes very important, in part, due to intense glutamate reuptake in astrocytes, that extracellular glucose levels are insufficient to sustain such uptake (1). In such a situation, glycogen present in the astrocyte is mobilized to provide the necessary glycosyl units (2) as previously demonstrated in vivo [17]. Glycolysis is the predominant pathway (3) and lactate is produced (4) to maintain the high glycolytic rate. Resynthesis of glycogen will cause additional glucose uptake that might contribute to create a mismatch between glucose utilization and oxygen consumption, a phenomenon known as “uncoupling” [18]. Source: Recreated with permission from Pellerin L, Bouzier-Sore AK, Aubert A, Serres S, Merle M, Costalat R, et al. Activity-dependent regulation of energy metabolism by astrocytes: an update. Glia 2007;55(12):1251–2.
In the absence of glutamatergic activation (Fig. 9.1), glucose is taken up by astrocytes via the glucose transporter GLUT1. Some of this glucose will undergo oxidative metabolism via glycolysis and the TCA cycle in the mitochondria to produce ATP and reducing equivalents. Some glucose, however, may be converted into lactate and released from the astrocyte through monocarboxylate transporters, MCT1 and MCT4. Once in the extracellular space, lactate is taken up by neurons via MCT2 in parallel with glucose. Pyruvate produced from both glucose and lactate can then be used oxidatively to provide neurons with the required resting energy needs.
Upon glutamatergic activation (Fig. 9.2), glutamate released from the presynaptic neuron binds to and activates AMPA and NMDA receptors on the postsynaptic neuron, allowing for the entry of sodium and other cations. After excitatory postsynaptic potentials are generated, the sodium-potassium ATPase (Na+/K+-ATPase) acts to reestablish the resting ion gradient, which requires substantial energy expenditure. In neurons, oxidative phosphorylation in the mitochondria is activated to replenish ATP, but this is done at the cost of NADH. To replenish NADH, TCA cycle activity is enhanced. As pyruvate (obtained from glucose through the process of glycolysis) enters the TCA cycle and the cytoplasmic levels drop, conditions inside the neuron become favorable for the use of both glucose and lactate. Interestingly, real-time epifluorescence microscopy studies show that glutamate may stimulate glucose uptake in astrocytes while simultaneously inhibiting its uptake in neurons [10]. Thus, it is possible that during glutamatergic activation, neurons prefer lactate as an oxidative substrate.
After glutamatergic activity, astrocytes can take up the glutamate from the synaptic cleft through glutamate transporters. This is accompanied by an influx of sodium ions, thus activating the Na+/K+-ATPase on astrocytes. In turn, aerobic glycolysis of glucose to pyruvate is increased, causing an initial rise in cytosolic NADH. This is normalized by the conversion of pyruvate to lactate by LDH, which can be released through MCT1 and MCT4. The now extracellular lactate can be taken up by neurons to meet additional energy demands, provide reducing equivalents to neurons [19], or used to replenish the extracellular pool. Further support of the ANLS model comes from the recent finding that inhibition of LDH hyperpolarized neurons and reduced seizures and epileptiform activity; neuron hyperpolarization could be reversed with pyruvate [20]. In fact, reducing circulating glucose concentrations by switching to a ketogenic diet (a diet consisting of mostly fat with minimal carbohydrates) has gained support as a therapeutic method to treat epilepsy [21].
Upon prolonged stimulation, glycogen breakdown and mobilization from astrocytes may be necessary to provide supplemental energy for neurons. Although glycogen storage is relatively low in the CNS compared to other peripheral tissues, it is still the largest energy reserve in the brain. Glycogen storage is more prevalent in nonneuronal cells [22], namely astrocytes [23], and its metabolism can yield lactate and ultimately ATP under anaerobic conditions to meet the high energy demands of the brain. Glycogenolysis can be triggered by various neuromodulators including noradrenaline (NA), vasoactive intestinal peptide (VIP), monoamines, and adenosine [24–26]. Glycolysis and glycogenolysis are thought to be activated successively after intense neuronal stimulation occurs. In this sense, glycogenolysis is sometimes viewed as an extension of the ANLS model.
The concept of the ANLS has gained a great amount of support over the years, but its acceptance is still controversial [27–31]. Although it is widely accepted that the brain can consume lactate during hypoglycemia and hyperlactemia [32], whether glucose or lactate is the primary energy source for neurons during neuronal activation is still debated. Criticisms of this model include evidence that both neurons and astrocytes have glucose transporters [29] and the importance of oxidative phosphorylation in energy production compared to the astrocyte-neuron lactate shuttle [28]. Recent evidence has demonstrated that the glucose oxidation in nerve terminals is substantial under both resting and activated conditions, suggesting that pyruvate from astrocytes would not be a major fuel source during neuronal activity [30]. It can be agreed, however, that glucose is and has always been the primary energy source in the brain but, under certain metabolic conditions, other energy sources such as lactate may be used.
Role of Energy Metabolism in Epilepsy
Seizures are the result of excessive synchronous neuronal activity in the brain and are associated with an increase in extracellular glutamate. The uptake of glutamate into glial cells induces a delayed, long-term stimulation of astrocytic glycolysis, which requires Na+/K+-ATPase activation [33]. Synaptic activity is accompanied by an acute fall in glucose concentration and is followed by a local surge in lactate concentration. During intense neuronal activity, the energy demand exceeds that of the glucose supplied from the bloodstream. Patients with epilepsy experience high levels of glucose uptake and hypermetabolism during seizures and low levels of glucose uptake and hypometabolism in between seizures [34]. Astrocytes contribute to the neuronal energy demand, potentially through the breakdown of glycogen storage.
Elevated levels of glycogen have been reported in the white matter, gray matter, and hippocampus of patients with temporal lobe epilepsy (TLE) [35]. Glycogen synthesis and storage occurs primarily in astrocytes because astrocytes have the glycogen synthesizing enzyme, glycogen synthase, whereas neurons do not. When energy is in high demand, astrocytes break down glycogen with the enzyme glycogen phosphorylase, and produce supplemental energy for neurons, potentially in the form of lactate to be shuttled through the ANLS. In mice injected with the glycogenic agent and convulsant methionine sulfoximine (MSO), brain glycogen increased in the preconvulsive state, was rapidly mobilized during seizures, and returned to high levels after seizures [36]. MSO is an inhibitor of glutamine synthetase (GS) [37], and a decrease in GS and consequently a decrease in the glutamate–glutamine–GABA shunt can result in decreased inhibitory transmission and reduced glutamate clearance from the extracellular space, perhaps accounting for the convulsant effects of MSO. Astrocytes play a major role in both brain metabolism and glutamate homeostasis, which may become dysregulated in epilepsy.
Glutamate Homeostasis in the Brain
Glutamate is the main excitatory neurotransmitter in the CNS. It is an L-amino acid with a carboxylic acid side chain that exerts its signaling role by acting on glutamate receptors found on cell membranes. Extracellular glutamate levels determine the extent of receptor stimulation; consequently, it is important to maintain low levels of glutamate in the extracellular space to ensure a high signal-to-noise ratio. Extracellular glutamate concentrations have been estimated to be between 1 and 30 μM, with the majority of studies finding levels below 5 mM [38,39]. Intracellular glutamate levels are several thousand fold higher, with the highest concentrations of glutamate found within nerve terminals [38]. During synaptic transmission, extracellular glutamate within the synapse reached levels above 1 mM and those levels decayed within milliseconds [40].
Accumulation of glutamate in the extracellular space can lead to cell excitotoxicity. Therefore, the brain must have a powerful protective system to maintain glutamate homeostasis in the brain. First, the blood-brain barrier (BBB) helps create an isolated environment that prevents circulating glutamate from entering the brain. Second, at synaptic clefts, neurons are surrounded by astrocytes that have such a high glutamate uptake activity that the healthy, noncompromised brain is remarkably resistant to glutamate toxicity. Third, a number of metabolic enzymes exist that allow for the synthesis and metabolism of glutamate. Finally, a well-established system between neurons and astrocytes, called glutamate–glutamine cycling, allows for the rapid uptake and conversion of glutamate into a nontoxic compound in astrocytes followed by its release into the extracellular space for uptake by neurons. All of these mechanisms are required to prevent neuronal excitotoxicity and all are possible with the aid of astrocytes.
Blood–Brain Barrier
Astrocytes play a major role in protecting the brain. In addition to controlling the chemical composition and levels of ions and nutrients in the extracellular space, astrocytes also modulate synaptic activity, store glycogen, and contribute to synapse formation and remodeling. Importantly, astrocytes help form and maintain the BBB through their cell projections called astrocytic endfeet. This barrier helps limit the net influx of glutamate into the brain, therefore allowing the brain to maintain an autonomous microenvironment unique to that of the rest of the body.
The BBB is a highly selective permeable barrier that separates the circulating blood from the extracellular fluid in the brain. It is formed by a layer of cerebral capillary endothelial cells connected by tight junctions that surround the CNS (Fig. 9.3) [43]. These cells separate the BBB into two barriers: (1) the luminal domain (membrane of the endothelial cells facing the blood) and (2) abluminal domain (membrane of endothelial cells facing the brain). Therefore, any molecules entering or leaving the brain must pass through two membrane barriers, each with distinct properties. Facilitative carriers exist exclusively on the luminal membrane while excitatory amino acid transporters (EAATs) exist only on the abluminal membranes. The EAATs couple Na+ transport with glutamate movement against the electrochemical gradient to move glutamate into the endothelial cells where it can diffuse into the blood via facilitative carriers [44]. This clever organization of the BBB allows for easy removal of glutamate from the brain extracellular fluid while preventing the net entry of serum glutamate into the brain. Thus, it promotes the maintenance of low extracellular glutamate concentrations in the brain. The role of the BBB in epilepsy will be discussed in Chapter 12: Blood–Brain Barrier Disruption.

The presence of Na+-dependent carriers capable of pumping glutamine and glutamate from brain into endothelial cells, glutaminase within endothelial cells to hydrolyze glutamine to glutamate and NH4+, and facilitative carriers for glutamine and glutamate at the luminal membrane provides a mechanism for removing nitrogen and nitrogen-rich amino acids from brain [41]. EAAT1, EAAT2, and EAAT3 are present in endothelial cells [42], and astrocytes, EAAT3 is present in nerve cells. A, Na+-dependent system A; N, Na+-dependent system N; EAAT, Na+-dependent glutamate transporter, xG–, facilitative glutamate transporter; n, facilitative glutamine transporter. Source: Reproduced with permission from Hawkins RA, O’Kane RL, Simpson IA, Vina JR. Structure of the blood-brain barrier and its role in the transport of amino acids. J Nutr 2006;136(1 Suppl):218S–26S.
Glutamate Transporters at the Synapse
In the CNS, a class of five excitatory amino acid transporters (EAAT1–5) are responsible for the synaptic reuptake of glutamate. The rodent homologs to EAAT1–5 are glutamate aspartate transporter (GLAST) glutamate transporter-1 (GLT1), excitatory amino acid carrier-1 (EAAC1), EAAT4, and EAAT5, respectively. GLAST/EAAT1 and GLT1/EAAT2 are predominantly expressed in astrocytes whereas EAAT3–5 are largely expressed in neurons. These transporters cotransport three Na+ and one H+ into the cell along with glutamate in exchange for the release of one K+. EAAT1–3 are efficient glutamate transporters with small associated macroscopic anion currents whereas EAAT4 and EAAT5 are low-capacity transporters that have a predominant anion (Cl−) conductance [45]. EAAT1 (GLAST) expression is most prominent in the cerebellum with only moderate levels in the forebrain whereas EAAT2 (GLT1) expression is most abundant in the forebrain and low within the cerebellum. EAAC (EAAT3) is expressed at low levels throughout the brain. EAAT4 is only found in the cerebellum whereas EAAT5 is only found in the retina.
Glutamate transporters play a major role in clearing glutamate from the extracellular space. At the tripartite synapse, the pre- and postsynaptic neurons are surrounded by astrocytes that abundantly express glutamate transporters. EAAT2 (GLT1) is responsible for the majority of glutamate clearance after synaptic activity and is essential in glutamate uptake. Although the cycle time of glutamate transport has been measured to be slow, ranging from 11–80 ms [38], it is possible that their highly efficient removal of glutamate from the extracellular space may be due to both their ability to quickly bind glutamate and their sheer abundance. Recently, it was discovered that even though GLT1 is anchored to the membrane at synapses, it can become untethered after glutamate is released into the extracellular space and can perform rapid, activity-regulated surface diffusion [46]. Therefore, GLT1 can readily move between synaptic and nonsynaptic sites to ensure glutamate clearance and effectively shape excitatory postsynaptic currents.
It is widely accepted that delayed clearance of extracellular glutamate is implicated in seizure development and spread [47–51]. During and Spencer [47] used microdialysis probes to record glutamate levels in the hippocampus before and during a seizure in human patients with complex partial epilepsy refractory to medical treatment. The dialysate concentration of glutamate rose before the occurrence of a seizure, which was followed by a sustained increase, potentially to neurotoxic levels, during a seizure in the epileptic hippocampus [47]. Elevated levels of extracellular glutamate have been reported in patients with various epilepsies, which could potentially be a consequence of altered expression or activity of glutamate transporters.
Glial Glutamate Transporters
Excitatory amino acid transporters, EAAT1 (GLAST) and EAAT2 (GLT1), are found throughout the CNS and are almost exclusively expressed on astrocytes. In fact, GLT1 protein is not expressed on neurons in the healthy adult CNS, however, mRNA encoding GLT1 has been found in some neurons [38]. GLT1 protein has been shown to be transiently expressed in several neuronal populations during development in the rodent and sheep brain, however, this expression disappears on maturation. GLAST is the dominant glutamate transporter in the cerebellum, expressed most densely on the Bergmann glia [52], and GLT1 is the predominant glutamate transporter in the forebrain, expressed most heavily in the hippocampus. GLAST levels are estimated to be 3200 and 18,000 per μm3 tissue in the young adult rat stratum radiatum in the hippocampus (CA1) and the cerebellar molecular layer, respectively [53]. The number of GLT1 in the young adult rat stratum radiatum of the hippcampus (CA1) was measured to be 12,000 per μm3 tissue and only 2800 per μm3 in the cerebellar molecular layer [53]. Through both human tissue studies and the use of animal models of epilepsy, researchers have begun to elucidate the role of glial glutamate transporters in the pathology of epilepsy.
Human Tissue Studies
Several immunohistological studies on human tissue from patients with TLE have characterized localization and expression changes of EAAT1 and EAAT2 in hippocampal sclerosis (HS). In the sclerotic hippocampus, EAAT2 immunoreactivity (IR) was markedly reduced in subfields exhibiting neuron loss, such as CA1, hilus, and CA4 [54,55]. In addition, an increase of EAAT1 IR was observed in CA2/3 stratum radiatum of the sclerotic tissue [55]. A small decrease in EAAT1 IR has been seen in the CA4 and in the polymorphic and supragranular layer of the dentate gyrus in HS [56].
Although studies have shown a decrease in EAAT2 immunohistological expression in HS tissue from patients with TLE [56], either no change [55] or an increase in EAAT2 IR has been reported in non-HS tissue. One study, however, did observe a change in localization of EAAT2 IR in TLE human tissue, but it was determined that this occurred postmortem [57]. Non-HS cases also showed no change in EAAT1 IR compared to autopsy controls [55]. Western blotting analysis of tissue from patients with TLE have shown slightly reduced EAAT1 levels [57] but no change in EAAT2 protein expression [57–59].
A number of studies have used in situ hybridization or northern blotting determine mRNA levels in tissue resected from TLE patients. In the nonsclerotic tissue, no statistically significant difference in EAAT1 mRNA was observed compared to control. EAAT2 mRNA signal intensity was decreased in all subareas compared to control hippocampus, but was only found to be significant in CA3 [56]. No significant change in EAAT1 mRNA and a significant reduction in EAAT2 mRNA levels were observed in the HS tissue [56]. In the hippocampal dentate granule cells, no change in EAAT2 mRNA was found [60]. One study did notice, however, a relative increase in mRNA content per cell in TLE compared to control tissue [57].
In studies examining the dysplastic tissue of human patients with focal cortical dysplasia (FCD), decreased IR and more diffuse pattern of EAAT1 and EAAT2 expression were observed [61,62]. No changes were found in EAAT2 mRNA in the epileptic foci compared to the nonepileptic regions of human neocortical epilepsy tissue, but EAAT2 protein expression was reduced [63]. Support of these findings was found in a freeze-induced rat model of cortical dysplasia. The nonselective glutamate transporter antagonist DL-threo-β-benzylozyaspartic acid (TBOA) prolonged postsynaptic currents (PSCs) and decreased the threshold for evoking epileptiform activity in the lesioned cortex compared to controls [64]. This effect could be mimicked with the specific GLT1 antagonist dihydrokainate (DHK), suggesting a role of GLT1 in regulating network excitability.
When examining human tissue data, it is important to keep in mind that tissue is often resected after patients have had epilepsy for prolonged periods and have become medically refractory. Therefore, results found are often the endpoint of a disease and may not provide information on disease progression. Animal models must be used to determine whether glial glutamate transporters are involved in epileptogenesis or are a consequence of epilepsy.
Animal Models
Glial glutamate transporters are essential in maintaining low extracellular glutamate concentrations. GLT1-deficient mice suffered from lethal spontaneous seizure due to the elevated extracellular glutamate levels in the brain [49]. Deletion [49] or antisense oligonucleotide-mediate inhibition of synthesis [65] of GLT1 in rodents revealed that it is the major contributor to glutamate uptake from the extracellular space. Intraventricular administration of GLT1 antisense oligonucleotide in rats resulted in a 32-fold rise in extracellular glutamate, measured by a microdialysis probe in the striatum. In addition, this treatment resulted in a progressive motor syndrome that included mildly paretic hindlimbs and dystonic posture [65]. Overexpression of GLT1 in transgenic mice, on the other hand, attenuated epileptogenesis and reduced chronic seizure frequency in a pilocarpine-induced model of epilepsy [66]. Without GLT1, glutamate levels rise enough to cause neurotoxicity and seizures whereas overexpression of GLT1 can offer neuroprotective effects.
Mice lacking GLAST show increased cerebellar damage after brain injury and motor discoordination [67]. In the amygdala kindling model, duration of generalized seizures was prolonged by about 35% in GLAST-deficient mice [68]. There was no significant difference, however, in afterdischarge threshold or in seizure responses induced by the first stimulation. GLAST-deficient mice also showed a shorter latency period and more severe stages of pentylenetetrazol (PTZ)-induced seizures compared to wild-type mice [68]. The inhibition of synthesis of GLAST by antisense oligonucleotides in rats resulted in a 13-fold rise in extracellular glutamate dialysate in the striatum and a progressive motor syndrome [65]. Like GLT1, GLAST plays a role in modulating extracellular glutamate levels and deletion of it may result in neurotoxicity.
Kainic acid (KA) is a cyclic analog of L-glutamate and a potent agonist for kainate receptors that can lead to status epilepticus (SE) in animals. Injection of KA into animals is characterized by a latent period followed by refractory spontaneous seizures, regardless of administration route [69]. It is now one of the most widely used animal models of TLE pathology. Injection of KA into the amygdala of rats led to the development of spontaneous limbic seizures within 14–25 days. Sixty days postinjection, both mRNA and protein levels of GLT1 and GLAST were downregulated in the ipsi- and contralateral hippocampus to injection [70]. In 7-week-old rats treated with intraperitoneal (i.p.) injections of KA, neuronal death was associated with focal loss of GLT1 IR in reactive astrocytes and stronger IR for glutamate in the thalamus [71]. This loss was observed between 14 and 28 days postinjection and mimicked the pathology seen in human tissue studies. In a subcutaneous injection of KA model of epilepsy in adult rats, in situ hybridization revealed that GLT1 mRNA levels transiently increased in the stratum oriens and radiatum of the hippocampus, as well as the molecular and polymorphic layers of the dentate gyrus [72]. GLAST hippocampal mRNA was increased 12 hours after systemic KA administration in a rat model of limbic seizures, peaked at 48 hours, and returned to baseline by 7 days postinjection [73].
Administration of pilocarpine, a muscarinic receptor agonist, into animals is commonly used as a model of mesial temporal lobe epilepsy (MTLE). Intraperitoneal injection of pilocarpine into 90-day-old rats led to a period of SE, which was then followed by a latent period of several days, until rats developed spontaneous seizures (chronic phase). GLT1 and GLAST protein and mRNA levels were downregulated in the cortex during the latent period. In the chronic period, GLT1 mRNA and protein levels were decreased in the hippocampus. Immediately after SE, however, no change in either GLT1 or GLAST was observed [74].
The kindling model of epilepsy, in which repeated electrical stimulations are given to an animal to induce seizures, may leave long-lasting effects on the brain. GLAST protein was downregulated in the piriform cortex/amygdala in kindled rats as early as 24 hours after a stage 3 seizure and persisted through multiple stage 5 seizures. No change, however, was observed in the hippocampus or limbic forebrain. GLT1 protein remained unchanged in any region examined (hippocampus, piriform cortex/amygdala, and limbic forebrain) after single or multiple seizure stimulations [75]. In situ hybridization analysis revealed no changes in GLT1 mRNA in the hippocampus or cortex of kindled rats that were sacrificed 28 days after the final stimulation [76]. An increase of GLT1 mRNA was observed, however, bilaterally in the striatum in kindled animals. Western blot analysis revealed no change in GLAST or GLT1 protein.
A number of genetic models of epilepsy exist, including the spontaneous epileptic rat (SER), which is a double mutant obtained by crossing tremor rats with zitter rats. In the 9–12-week-old SER, GLAST mRNA was reduced and GLT1 mRNA was mildly increased in the hippocampus. The number of GLAST-positive cells in the hippocampus of the SER was reduced compared to control rat, particularly in CA3 and the dentate gyrus. GLAST protein levels were slightly downregulated whereas GLT1 protein levels were substantially upregulated in SER hippocampus [77]. Reduction of GLT1 mRNA expression, but no change in protein levels, were seen in the inferior colliculus, cortex, striatum, and CA1 of genetically epileptic-prone rats 7 days after a series of audiogenic stimulus exposures [78].
A genetic model of TLE can be created using an inbred mutant strain of DDY mice. These animals experience complex partial seizures with secondary generalization. GLT1 mRNA and protein in the parietal cortex remained unchanged but levels in the CA3 hippocampus were reduced. GLAST protein was reduced in the hippocampus, while no changes in mRNA or protein were observed in the parietal cortex [79].
The genetic absence epilepsy rat from Strasbourg (GAERS) is considered an animal model of inherited human absence epilepsy. The use of in situ hybridization revealed increases in GLT1 mRNA levels in the ventromedial nucleus of the thalamus and the subthalamic nucleus while increased GLAST mRNA was found in the primary somatosensory cortex and temporal cortex of GAERS [80]. In another study, epileptic discharges were recorded in the thalamo–cortical network began around 40 days after birth in GAERS. Although no GLAST or GLT1 protein changes were observed in adult rats, 30-day-old GAERS showed a reduction in cortical GLT1 and GLAST protein [81]. No change in GLT1 and GLAST protein expression was observed in the thalamus or hippocampus. The expression and activity of GLAST were decreased by 50% in newborn GAERS cortical astrocytes grown in primary culture, which was accompanied by a reduction in glutamate uptake [81].
In a model of posttraumatic epilepsy (PTE) that involves ferrous chloride injections into the rat cortex, GLT1 cortical protein levels were downregulated in the epileptic rat 1 and 4 days after induction, but not at 3 months. GLAST cortical protein expression, however, remained unchanged [82]. After injection of Fe3+ into the left amygdala, rats experienced spontaneous limbic behavioral seizures. In this model, GLT1 protein increased 5 and 15 days after iron injection on the contralateral side to injection and returned to basal levels by 30 days. GLAST protein showed an initial increase but the protein was persistently downregulated by 15 days postinjection [83].
A number of neurological disorders are accompanied by epilepsy. For example, in rats with cerebral ischemia-related epilepsy, GLT1 immunohistochemical staining was decreased in hippocampal CA1 and in the motor cortex compared to rats with cerebral ischemia without epilepsy [84]. In the tuberous sclerosis (TSC) epilepsy model in which the astrocyte-specific Tsc1 gene is inactivated, mice exhibit decreased GLT1 and GLAST protein expression and decreased glutamate transport currents, suggesting that these glial glutamate transporters contribute to the glutamate dysregulation and seizures in TSC [85]. Sheepdogs with familial idiopathic epilepsy have reduced GLT1 immunostaining, particularly in the cerebral cortex and lateral nucleus of the thalamus, whereas there was no difference in GLAST immunolabeling compared to control dogs [86].
Taken together, these results strongly support the notion that astrocytes play a crucial role in protecting neurons from hyperexcitability and glutamate excitotoxicity. Elevated extracellular glutamate levels play a role in seizure generation and spread. Current literature on animal models and human tissue studies suggest a dysregulation of EAAT1/GLAST and EAAT2/GLT1 in certain epilepsies, however, whether dysregulation or dysfunction of glial transporters plays a causative role in epilepsy still remains unclear.
Neuronal Glutamate Transporters
EAAT3 (EAAC1) concentration is lower than that of the other EAATs and is mostly expressed in neurons. Unlike GLT1 and GLAST, EAAC1 is mostly intracellular and can transport cysteine. It is most abundantly expressed in the hippocampus, cerebellum, and basal ganglia [38] and is found in a variety of neurons. Although it is predominantly expressed by neurons in the healthy CNS, EAAC1 has also been found in oligodendrocytes and, in the injured brain, in microglia [87]. Administration of EAAC1 antisense oligonucleotide in rats has been shown to produce mild neurotoxicity and seizures in less than a week of treatment [65].
The expression of EAAT4 is predominantly found in the cerebellum, but low levels have been detected in the forebrain. It is most extensively found on the Purkinje cell plasma membrane and the highest levels are found perisynaptically [88]. The average concentration of EAAT4 in the molecular layer of adult rats is estimated to be 1900 molecules per μm3 tissue. Even though EAAT4 shares a similar mechanism to EAAT1-3, it operates 5–10 times more slowly [89]. Unlike EAAT1-3, both EAAT4 and EAAT5 are highly associated with Cl− conductance. EAAT5 is only found in the retina and is considered a low-capacity and low-affinity glutamate transporter [90]. Although reduced levels of EAAT4 mRNA and protein has been found in human epileptic neocortex tissue [63], EAAT5 has not been implicated in epilepsy and therefore will not be discussed further in this chapter.
Human Tissue Studies
Hippocampal neuronal loss is commonly observed in human TLE patients. Despite this, EAAT3 IR was increased in HS on pyramidal [60] and remaining granule cells [55,60]. In a separate study, fewer EAAT3 positive cells were found in the sclerotic hippocampus than in the non-HS in patients with pharmaco-resistant TLE. Individual neurons, however, showed an increase in EAAT3 IR in both HS and non-HS [56], although no differences between non-HS EAAT3 IR and autopsy control has also been reported [55]. The use of in situ hybridization revealed that EAAT3 mRNA did not change in non-HS tissue, but did decrease in the sclerotic hippocampus in CA4 compared to nonepileptic control tissue [56].
In neocortical tissue resected from patients with cortical dysplasia-induced medically intractable focal epilepsy, an increase of EAAT3 immunohistochemical staining was observed [60,62]. Northern blotting revealed elevated EAAT3 mRNA in dysplastic neurons in cortical dysplasia compared to postmortem control [60]. The localized epileptic foci showed reduced mRNA and protein levels of EAAT3 and EAAT4, assayed by real-time PCR and Western blot, respectively, compared to the nonepileptic regions in human neocortical epilepsy tissue [63].
Animal Models
The neuronal glutamate transporter EAAC1 is in lower abundance than GLT1 and GLAST and only plays a minor role in glutamate uptake. Despite this, EAAC1 has been implicated in epilepsy. Antisense oligonucleotide inhibition of EAAC1 in rats did not result in elevated extracellular glutamate in dialysate from the striatum, but it did produce mild neurotoxicity and resulted in spontaneous, recurrent seizures [65]. Mice deficient in EAAC1, however, were not more susceptible to PTZ-induced seizures compared to wild-type mice [91]. A variety of epilepsy models have been used to elucidate the role of EAAC1 in epilepsy.
Striking changes in EAAC1 IR have been observed in both the KA and pilocarpine models of epilepsy. An analysis of the various layers of the hippocampus revealed specific changes in EAAC1 IR in the subcutaneous KA-induced epilepsy model in rats. Reduced EAAC1 IR in the CA1 stratum lacunosum moleculare layer was observed within 4 hours after seizure onset [72] and continued to drop until it was almost completely eliminated by 5 days posttreatment. A transient increase of EAAC1 immunostaining in the dentate granule cells was observed 4 hours post-SE after KA treatment [72], while an increase in EAAC1 IR in the dentate granule cells was seen weeks after pilocarpine-induced seizures [60]. A decrease in EAAC1 IR in CA1 and CA3 pyramidal cells of the hippocampus was observed 5 days after KA administration. No immunohistological changes in EAAC1 IR were seen in the cerebral cortex or in the corpus callosum after KA-induced epilepsy [72].
Changes in EAAC1 IR often paralleled similar mRNA dysregulation. Decreased mRNA levels in CA1 and CA3 pyrimadal cells were seen as early as 4 hours after subcutaneous KA treatment and persisted out to 5 days. A consistent decrease in EAAC1 mRNA in the dentate gyrus up to 8 hours post KA-induced seizures was followed by a transient increase 16 hours after treatment before levels returned to baseline [72]. Similarly, an increase in EAAC1 mRNA levels in the dentate granule cells of rats was observed in the pilocarpine model of TLE 2 weeks after seizure induction [60]. In the amygdala KA model in rats, hippocampal EAAC1 mRNA levels were increased 60 days after injection, but protein levels were unchanged [70]. Western blot analysis of EAAC1 in the amygdala-kindled rat model showed increased levels of protein in the piriform cortex/amygdala and hippocampus after 24 hours in animals that reached stage 5 seizures, but no change was observed in the limbic forebrain [75].
SERs exhibit sporadic tonic convulsions and absence-like seizures, similar to those seen in humans. In both SER [77] and 4-month-old GAERS [81], hippocampal EAAC1 mRNA and protein levels showed no significant difference when compared to control mice. The 4-month-old GAERS also showed no change in EAAC1 mRNA and protein levels in the thalamus. In the cortex, however, mRNA levels were increased in 4-month-old GAERS, but no change in protein levels were found in either the 30-day- or 4-month-old animals [81]. In addition, no changes in hippocampal EAAC1 protein, but decreased levels of thalamic protein levels, were observed in the 30-day-old GAERS.
Various other epilepsy models showed significant increases in EAAC1 expression. Injections of Fe3+ into the rat amygdala causes chronic, spontaneous recurrent focal seizures with generalized limbic behaviors. In this model of epilepsy, rats experienced a bilateral increase in EAAC1 protein expression for up to 30 days after injection [83]. The peak of EAAC1 expression, however, was 5 days postinjection. In a rat model of global cerebral ischemia induced by chest compressions, 64% of rats developed epilepsy. Those who did exhibited increased EAAC1 IR in hippocampal CA1 and the motor cortex compared to ischemic mice who did not develop epilepsy [84].
Alterations in EAAT3/EAAC1 are clearly prominent in a variety of human tissue studies and in a number of different epilepsy models. Increased IR was commonly observed in epileptic human tissue, however, this was not always paralleled by animal studies. Looking at different time points and brain regions in animal epilepsy models revealed that changes in EAAC1 expression are not static but, in fact, are constantly changing. In comparison to EAAT1–3, EAAT4 and EAAT5 are not well studied in epilepsy. Functional assays of glutamate transporters will be helpful in future studies to determine what role these proteins play in epileptogenesis.
Metabolic Enzymes
Since little to no glutamate can enter the brain through the BBB, glutamate must be synthesized and metabolized within the CNS. A number of metabolic enzymes help regulate glutamate homeostasis in the brain. Overall mechanism, cellular distribution, and localization of a subset of these enzymes are listed in Table 9.1. Once glutamate is taken into the cell, it can be metabolized in a number of ways (Fig. 9.4) [92].
Table 9.1
Key Regulatory Enzymes in Glutamate Metabolism
Enzyme Name | Reaction Catalyzed | Predominant Cell Type | Intracellular Localization |
Alanine aminotransferase (ALT) | Glutamate +pyruvate ![]() | Astrocytes Neurons | Mitochondria Cytoplasm |
Aspartate aminotransferase (AST) | Aspartate +α-ketoglutarate ![]() | Neurons | Cytoplasm Mitochondria |
Glutamate Decarboxylase (GAD) | Glutamate +H2O ![]() | Neurons | Cytoplasmic vesicles |
Glutamate dehydrogenase (GDH) | Glutamate +NAD(P)+![]() | Astrocytes Neurons | Mitochondria |
Glutaminase | Glutamine +H2O ![]() | Astrocytes Neurons | Mitochondria |
Glutamine Synthetase (GS) | Glutamate +NH3 +ATP ![]() | Astrocytes | Cytoplasm |
Cytosolic Malic Enzyme (ME) | Malate +NADP+ ![]() | Astrocytes | Cytoplasm |
Pyruvate Carboxylase (PC) | Pyruvate +CO2 +ATP +H2O ![]() | Astrocytes | Mitochondria |
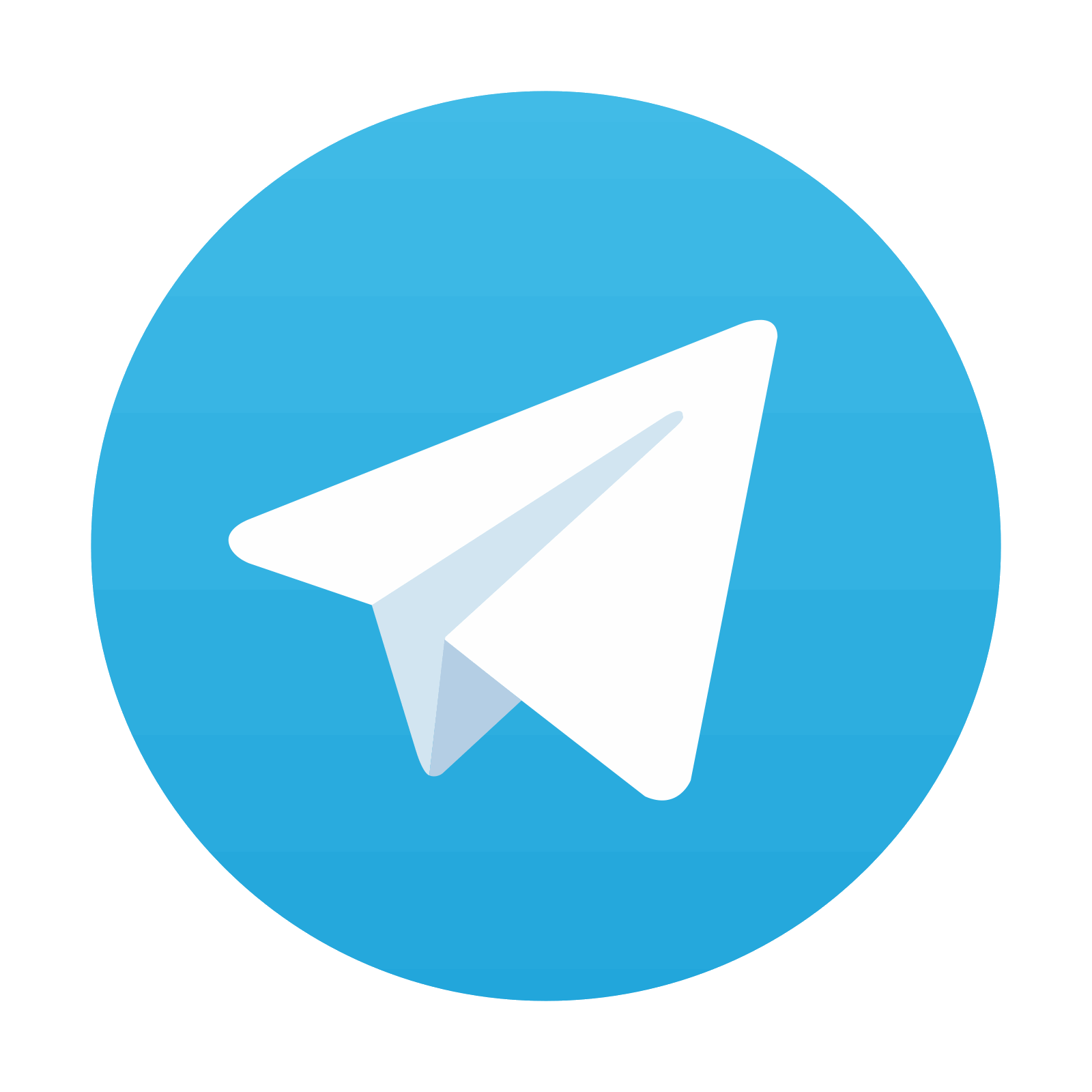
Stay updated, free articles. Join our Telegram channel
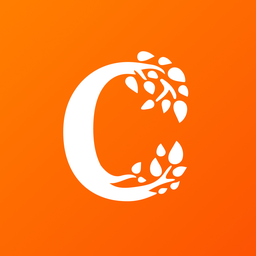
Full access? Get Clinical Tree
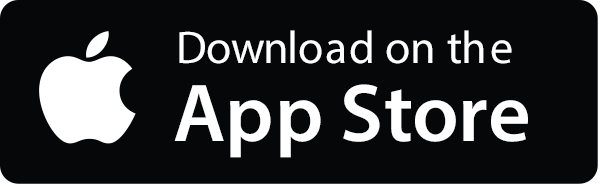
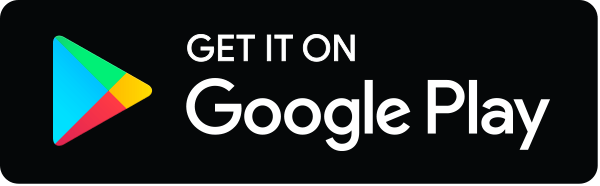
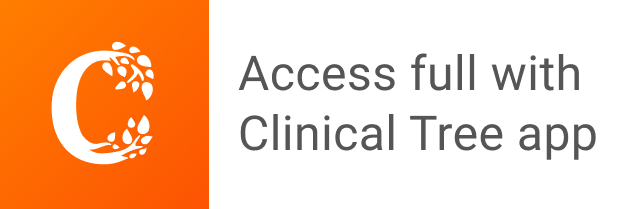