Keywords
Glycogen, polyglucosan, rhabdomyolysis, weakness, cramps, enzyme replacement therapy (ERT)
Introduction and Background
Metabolic myopathies are clinical disorders in which defects of adenosine triphosphate (ATP) production cause muscle dysfunction. A review of muscle metabolism is beyond the scope of this chapter and readers are referred to other reviews for further details. Nevertheless, a brief description of the biochemical pathways supporting the energy requirement of skeletal muscle is necessary to understand the symptoms of patients with metabolic myopathies, including disorders of glycogen metabolism.
Skeletal muscle is highly energy dependent and therefore vulnerable to disorders of energy metabolism. The hydrolysis of ATP to adenosine diphosphate (ADP) and inorganic phosphate (Pi) is the immediate and essential source of energy for contraction and relaxation. In order to regenerate ATP, skeletal muscle utilizes different substrates (high-energy phosphate compounds, glucose and glycogen, and free fatty acids [FFAs]), and different metabolic pathways (the creatine kinase (CK) reaction; anaerobic glycolysis; the β-oxidation spiral; oxidative phosphorylation).
Resting muscle utilizes predominantly FFAs (see Chapter 40 ). In working muscle, the bioenergetic pathway is dictated by the type, intensity, and duration of exercise.
Anaerobic glycolysis and the creatine kinase reaction, the major anaerobic sources of ATP, are important fuel sources in ischemic or isometric exercise, when muscle blood flow and oxygen delivery to exercising muscles are interrupted or reduced, and during extremely intense physical activity, when the energy demand is above the anaerobic threshold. During isotonic exercise, such as walking or running, ATP is regenerated by high-energy phosphate compounds during the first several minutes, after which carbohydrate oxidation increases and glycogen becomes the most important fuel. With prolonged exercise, glucose and glycogen stores are depleted, and there is a metabolic switch to fatty acid metabolism. After a longer period of exercise, FFAs are the predominant sources of ATP. Except for anaerobic glycolysis, the final common pathway for ATP production is the mitochondrial respiratory chain (oxidative phosphorylation; see Chapter 41 ) ( Figure 39.1 ).

Defects in ATP production cause clinical disorders characterized by muscle symptoms, which will depend on the specific metabolic pathway that is impaired. For example, defects of glycolysis will be evident after short intervals of moderate to intense exercise, whereas fatty acid metabolism disorders will cause symptoms after prolonged exercise.
This chapter reviews those disorders of glycogen metabolism (glycogenoses) that affect skeletal muscle alone, or in combination with other tissues ( Figure 39.2 ). These disorders include three enzyme defects involving nonlysosomal glycogenolysis and one affecting lysosomal glycogenolysis, seven defects of glycolysis, and three of glycogen synthesis.

Glycogenoses cause two main clinical syndromes :
- 1.
Exercise intolerance, with cramps, and myoglobinuria: these are generally associated with defects of glycogenolysis (phosphorylase kinase and myophosphorylase deficiencies) and glycolysis (phosphoglucomutase, phosphofructokinase, aldolase, beta-enolase, phosphoglycerate kinase, phosphoglycerate mutase, and lactate dehydrogenase deficiencies).
- 2.
Progressive weakness involving limb and trunk muscles: this is characteristic of defects in the glycogenosynthetic pathway (glycogenin and brancher enzyme deficiency) and in the lysosomal glycogenolytic system (acid maltase deficiency).
However, there are exceptions: (1) Defects of debrancher, a glycogenolytic enzyme, usually cause weakness rather than cramps and myoglobinuria; (2) patients with myophosphorylase or phosphofructokinase deficiency often develop fixed weakness later in life; (3) some patients with deficiencies of myophosphorylase, phosphorylase kinase, or phosphofructokinase present with weakness, without cramps and myoglobinuria; and (4) conversely, exercise intolerance characterizes a defect of glycogen synthesis, glycogen synthetase deficiency.
All glycogenoses are autosomal recessive, except phosphoglycerate kinase, which is X-linked, and phosphorylase kinase, which may be either. All of the genes encoding these enzymes have been cloned, their chromosomes have been localized, and many molecular genetic defects have been identified.
Forearm Ischemic Exercise Test
The forearm ischemic exercise test (FIET) is a useful tool to corroborate the diagnosis of defects in the glycogenolytic or glycolytic pathways. The test measures basal and post-exercise venous lactate and ammonia levels. In normal adults, lactate increases 3- to 5-fold over baseline with a peak at 1 to 2 minutes post-exercise, whereas ammonia increases 5- to 10-fold. Patients with myophosphorylase, phosphofructokinase, or distal glycolytic enzyme deficiencies generally show absent or inadequate (less than 1.5-fold) increases of lactate and exaggerated rises in ammonia. Conversely, a normal lactate response with impaired ammonia production is characteristic of myoadenylate deaminase deficiency.
However, the test has several limitations. The rise of venous lactate depends on the subject’s ability and willingness to exercise vigorously. The simultaneous measurement of ammonia levels is therefore important in order to confirm the adequate effort of the patient. Furthermore, affected individuals can develop muscle cramping and prolonged contractures, and should stop exercising at the first appearance of cramping to reduce the risk of muscle necrosis. Because of these reasons, the test is rarely performed in children.
To avoid pain and contractures, the test has been performed in 30 children with suspected muscle glycogenoses under semi-ischemic conditions, allowing systolic blood flow. Using this modification, all subjects were able to exercise for the whole minute without problems, and two patients with a defect in the glycolytic pathway failed to adequately increase venous lactate but did not experience contractures during exercise.
Furthermore, it has been shown that maximal intermittent handgrip exercise for one minute without ischemia provides a stimulus strong enough to elicit maximal glycolytic flux. Ischemic and non-ischemic tests were performed in eight patients with McArdle disease and in eight matched healthy control subjects. The non-ischemic test had the same diagnostic power for McArdle disease as the ischemic test but, unlike the ischemic test, did not cause muscle cramps or pain to the patients. Therefore, a non-ischemic forearm exercise test is an easy, safe, and well-tolerated tool in the diagnostic investigation of disorders of muscle carbohydrate metabolism.
Defects of Glycogenolysis
Phosphorylase Kinase Deficiency
Phosphorylase b kinase (PhK) is a regulatory enzyme in the activation cascade of glycogenolysis. By phosphorylating and thus activating glycogen phosphorylase, PhK stimulates glycogen degradation in response to various neural and hormonal signals.
The enzyme is a decahexameric protein composed of four subunits (α,β,γ,δ). Subunits α and β are regulatory, γ is catalytic, and δ is identical to calmodulin. Two isoforms are known for subunit α (muscle and liver, α M and α L ) and for subunit γ (muscle and testis, γ M and γ T ). The genes encoding α Μ ( PHKA1 ) and α L ( PHKA2 ) are both on the X chromosome, whereas the β ( PHKB ), γ M ( PHKG1 ), and γ T ( PHKG2 ) genes are autosomal.
PhK deficiency is associated with four different phenotypes, distinguished by tissue involvement (liver, muscle, liver and muscle, heart), and mode of inheritance (X-linked or autosomal).
- 1.
Liver disease, the most frequent type, is usually a benign condition of infancy or childhood, characterized by hepatomegaly, growth retardation, delayed motor development, and fasting hypoglycemia. Inheritance is X-linked recessive, due to mutations in PHKA2 . The enzyme defect is also expressed in erythrocytes and leukocytes, but not in muscle.
- 2.
Liver and muscle disease is characterized by hepatomegaly, usually resolving with age, and nonprogressive myopathy. Transmission appears to be autosomal recessive in most cases and due to mutations in PHKB . The enzyme defect is expressed in muscle and liver.
- 3.
Myopathy, inherited as an autosomal recessive or as an X-linked recessive trait, has been reported in about 20 cases. The clinical picture consists of exercise intolerance, with cramps, myalgia, and weakness of exercising muscle. Myoglobinuria after strenuous exercise has been described in less than 50% of the cases. Onset is usually in childhood or adolescence. Although fixed weakness was only present in a few cases, one of the patients reported by Clemens and associates, a 58-year-old man, had progressive weakness starting at age 46 and affecting distal more than proximal muscles. In most patients, serum CK level is variably increased.
The FIET is often normal or only mildly altered, and electromyography (EMG) can be normal or show nonspecific myopathic changes. Muscle biopsies may be normal or show subsarcolemmal accumulation of glycogen. Although PhK activity in muscle homogenate is absent or markedly decreased, the generally mild presentation of this myopathy has raised the question of whether this is indeed a disease or merely a metabolic variant.
- 4.
Cardiomyopathy has been described in a few infants and is well defined in terms of clinical pathology and biochemistry. The clinical phenotype is uniformly severe and survival has not exceeded months. The isolated involvement of the heart was a riddle because there is no cardiac isoform of PhK. Now we know that the PhK deficiency in these cases is secondary to de novo mutations in the gene ( PRKAG2 ) encoding the γ2 subunit of the AMP-activated protein kinase (AMPK).
Myophosphorylase Deficiency
Muscle phosphorylase (PYGM) initiates glycogen breakdown by removing 1,4-glucosyl residues phosphorolytically from the outer branches of the glycogen molecule, with liberation of glucose-1-phosphate: this goes on until the peripheral chains are shortened to about four glucosyl units. The degraded polysaccharide is known as phosphorylase-limit dextrin (PLD), and its short peripheral chains are removed by the debranching enzyme. In muscle, phosphorylase exists in two forms: a more active, phosphorylated a form, and a less active, dephosphorylated b form. The phosphorylation of myophosphorylase is catalyzed by PhK.
Human phosphorylase has three isozymes, muscle (PYGM), liver (PYGL), and brain/heart (PYGB), encoded by different genes that have been mapped on different chromosomes. Normal adult human muscle expresses only the muscle isozyme, whereas heart and brain express, in different amounts, both the brain and the muscle isoforms. In particular, heart contains the muscle isozyme (13%), the brain isozyme (58%), and a hybrid of the two isozymes (29%). Brain contains the muscle isozyme (8%), the brain isozyme (64%), and a hybrid of the two (28%), whereas liver contains exclusively the liver isozyme. Therefore, patients with the muscle isozyme deficiency must also have partial defects in heart and brain; however, symptoms of heart or brain involvement have not been reported.
A defect of the muscle isoform underlies glycogenosis type V (McArdle disease), whereas phosphorylase deficiency in liver is referred to as glycogenosis type VI (Hers’ disease).
McArdle disease is characterized by exercise intolerance with premature fatigue, myalgia, and cramps (see Case Example 39.1 ). Symptoms usually occur during brief and intense exercise (pushing or lifting heavy objects) or during sustained, but less intense exercise (climbing stairs). Most patients experience the so-called second-wind phenomenon: if they rest briefly at the onset of symptoms, they can resume exercising without discomfort. Myoglobinuria occurs in about 50% of patients and half of these develop renal failure. Although exercise intolerance is present from childhood, cramps and myoglobinuria develop later in life, and the diagnosis is usually established in young adult life.
A 16-year-old Caucasian boy presented with exercise intolerance since early childhood. Any attempt to perform vigorous exercise caused painful shortening of the muscles that were engaged in the activity (e.g. arms and shoulder muscles after shoveling snow), which resolved with rest. He also noticed that, if he paused at the first appearance of muscle stiffness, he was able to resume activity with more ease. He never noted pigmenturia.
Physical and neurologic examinations were unremarkable. Resting serum CK level was slightly elevated (468 U/L, normal<150). A FIET caused no increase of venous lactate above baseline, but normal rise in ammonia levels. Electrophysiologic studies were noncontributory. A muscle biopsy specimen (vastus lateralis) showed excessive variation of fiber size, subsarcolemmal deposits of glycogen by periodic acid–Schiff (PAS) staining, and negative histochemical reaction for phosphorylase. Biochemical analysis showed complete absence of phosphorylase activity whereas activities for phosphofructokinase and other glycolytic enzymes were normal. Molecular studies of genomic DNA extracted from blood showed that the patient was homozygous and his parents heterozygous for the common R50X nonsense mutation.
Comment
The symptoms in this young man (cramps induced by vigorous exercise) are typical—but certainly not specific—of McArdle disease. The description of a second wind phenomenon bolstered the diagnosis of McArdle and suggested looking for the common PYGM mutation in blood cells, which confirmed the diagnosis already established by muscle histochemistry and biochemistry.
Although the clinical phenotype is rather uniform, a few variants have been reported, including a mild form with excessive tiredness and poor stamina, a late-onset form with fixed weakness in the fifth to sixth decade without cramps or myoglobinuria, and a rare fatal infantile form characterized by weakness, severe respiratory insufficiency, and early death. Congenital myopathy and mental retardation were reported in a 4-year-old boy.
Laboratory tests show increased resting serum CK, flat venous lactate response to ischemic exercise, and myopathic EMG. The diagnosis is confirmed by the histochemical or biochemical documentation of phosphorylase deficiency in muscle and by molecular analysis of DNA. The presence of subsarcolemmal or intermyofibrillar deposits of glycogen gives muscle a vacuolar appearance ( Figure 39.3A,B ).

In patients with myophosphorylase deficiency, phosphorylase activity is normal in erythrocytes, leukocytes, platelets, and fibroblasts. Therefore, the diagnosis relies on measurement of phosphorylase activity in muscle or on genetic testing.
The histochemical reaction for phosphorylase is a valuable diagnostic tool because in most patients muscle fibers have no enzyme activity ( Figure 39.3D,E ). However, the phosphorylase reaction can be positive in rare cases with some residual enzyme activity and in biopsy specimens with abundant regenerating fibers. This false-positive reaction, which is due to the expression of PYGMB, may be misleading in muscle biopsy specimens taken too soon after an episode of myoglobinuria, when regeneration is at its most active.
The myophosphorylase gene has been cloned, assigned to chromosome 11q1, and its genomic structure has been described. The disease is transmitted as an autosomal recessive trait, but pseudo-dominant transmission has been documented in a few families. The molecular heterogeneity of myophosphorylase deficiency is striking: in the 20 years from the description of the first molecular defects, more than 100 different mutations have been reported. The most common mutation in North America and Northern Europe is the R50X. This has allowed the use of molecular genetic analysis in leukocytes for diagnostic purposes, thus circumventing the need for a muscle biopsy. However, as leukocytes are increasingly used for diagnosis, it becomes important to keep in mind the relative frequency of distinct mutations in different ethnic groups. For example, the R50X mutation has never been observed in Japan, where the most common mutation appears to be a 3-bp deletion, TTC at codon 708/709. The genotype/phenotype relationship in McArdle disease remains unclear. For example, the common R49X mutation was also found in an infant with the fatal variant, in a child who died of sudden infant death syndrome, and in two virtually asymptomatic children with persistent and unusually high levels of serum CK.
The exercise intolerance in McArdle disease is due to two main mechanisms. First, the block of anaerobic glycolysis deprives muscle of the energy required for isometric exercise. Second, the block of aerobic glycogen utilization and the consequent shortage of pyruvate and acetyl-CoA impairs dynamic exercise above a certain intensity (about 50% VO 2max ). The impairment of oxidative phosphorylation is evidenced by the decrease in oxygen extraction and maximum oxygen uptake documented in patients with myophosphorylase deficiency, which can at least partially be restored by intravenous glucose infusion.
There is no specific therapy for McArdle disease, but regular moderate aerobic training is effective because it favors alternate fuel delivery and utilization. In cases with residual phosphorylase activity, vitamin B6 should be tried because the overall body stores of pyridoxal phosphate (PLP) are depleted in McArdle disease due to the frequent lack of enzyme protein (to which PLP is bound ). Patients should be warned about the risks of strenuous exercise and advised to seek medical attention at the first appearance of pigmenturia, especially if accompanied by oliguria. Sucrose ingestion before exercise is beneficial but may lead to weight gain.
The two spontaneous animal models of McArdle disease—Charolais cattle and Merino sheep —are not practical for experimentation. However, a knock-in mouse model of the R50X mutation recapitulates faithfully the human disease and will provide valuable information on pathophysiology and experimental therapy.
Debranching Deficiency
Debranching enzyme is a monomeric protein encoded by a single gene and expressed ubiquitously in all tissues. It has two independent catalytic activities: transferase and α-glucosidase. After phosphorylase has shortened the peripheral chains of glycogen to about four glucosyl units, these residual stubs are removed by the debranching enzyme in two steps, catalyzed by an oligo-1,4-1,4-glucantransferase and by an amylo-1,6-glucosidase. The transferase and glucosidase activities can be assessed separately or together by using appropriate substrates.
Debrancher deficiency (glycogenosis type III) typically presents as a childhood-onset liver disease with hepatomegaly, growth failure, fasting hypoglycemia, and, less frequently, hypoglycemic seizures. Liver symptoms improve with age and often resolve completely around puberty.
Although clinical cardiopathy is infrequent, cardiac involvement is demonstrable by laboratory tests in most patients with myopathy and a child was reported to have died of cardiac failure at 4 years of age.
Myopathy is not always manifest in infants or children, who may show hypotonia, delayed motor milestones, or mild weakness. Myopathy often appears in adult life, long after liver symptoms have remitted or in patients without any history of hepatopathy. However, in 7 of 22 patients reviewed by Cornelio and colleagues onset was in childhood and the diagnosis was facilitated by the coexistence of liver disease. Two of these children had exercise intolerance, cramps, and premature fatigue, and five had diffuse weakness and wasting, growth retardation, and delayed motor development.
Adult-onset myopathies can be predominantly distal or generalized. Patients with distal myopathy develop wasting of distal leg and intrinsic hand muscles, often leading to the diagnosis of motor neuron disease or peripheral neuropathy: the “mixed” myopathic and neurogenic EMG pattern and the often slowed nerve conduction velocity suggests that weakness in these patients may have a neurogenic component. Patients with generalized myopathy tend to have more severe weakness, often affecting respiratory muscles.
It may seem paradoxical that the typical presentation of debrancher deficiency is so different from that of McArdle disease, although the two defective enzymes act sequentially in the first step of glycogenolysis. However, cycle ergometry with or without glucose infusion in six young patients with debrancher deficiency and no or only mild proximal weakness has documented that peak oxygen consumption was below normal and glucose improved work capacity by lowering heart rate and increasing peak work rate. Thus, even before developing overt weakness, patients with debrancher deficiency have exercise intolerance, not unlike patients with McArdle disease, albeit to a much lower extent.
Muscle biopsy specimens typically show large PAS-positive vacuoles containing diastase-digestible glycogen under the sarcolemma and between myofibrils ( Figure 39.4A,B ). Ultrastructurally, the vacuoles are collections of free and apparently normal glycogen β-particles ( Figure 39.4C ).

The gene encoding human debrancher ( AGL ) has been cloned, sequenced, and assigned to chromosome 1p2, and several different mutations have been identified.
Although there is no specific therapy for debrancher deficiency, various interventions, including dietary regimens, liver transplantation, physical therapy, and precautions with anesthesia and pregnancy, are discussed in a review article.
A Pediatrician’s Perspective
The disorders of glycogenolysis described earlier, and especially those affecting muscle, tend to affect adults more than children. However, the following exceptions are worthy of note.
The “liver and muscle” variant of PhK deficiency is typically seen in childhood. An interesting phenomenon is illustrated by a large family that we reported in 1985. Seven male children had an X-linked disorder characterized by hepatomegaly, hypotonia, and weakness in childhood (but resolving with age), delay in growth and sexual maturation, and gouty arthritis. Glycogen storage was severe in liver but also present in muscle, both histochemically and biochemically. We considered PhK deficiency a likely diagnosis, but found normal enzyme activities in both liver and muscle. In 1997, molecular analysis in the propositus by Drs. Jan Hendrickx and Patrick Willems (University of Groningen, the Netherlands) revealed a pathogenic mutation in the gene encoding the liver α-subunit of PhK ( PHKA2 ). As these authors have documented, there are two subgroups of X-linked glycogenosis, XLGI and XLGII, distinguished by the fact that PhK deficiency is demonstrable in liver from patients with XLGI but not in liver from patients with XLGII. Mutational analysis has shown that molecular defects in XLGI affect the stability of the protein whereas those in XLGII affect the regulation of enzyme activity, which probably explains the normal PhK activity that we had observed in two tissues. Although hypotonia in these children can be attributed to liver dysfunction, weakness and glycogen storage in muscle are more difficult to explain. Irrespective of the exact pathogenesis, mutations in PHKA2 should be considered in the differential diagnosis of children with liver and muscle glycogenosis, even in the absence of enzyme deficiency.
Myophosphorylase deficiency (McArdle disease) is typically a disease of young adults and is usually diagnosed in children only when older siblings are affected. In addition to the rare fatal infantile variant, pediatricians should also remember that early onset of symptoms, including myoglobinuria, can be due to genetic “double-trouble”—that is, the coexistence of homozygous mutations in the myophosphorylase gene and in the gene encoding myoadenylate deaminase.
Defects of Glycolysis
Phosphoglucomutase Deficiency
Phosphoglucomutase (PGM) deficiency has been described thus far in a single patient, a 34-year-old man who had two episodes of exercise-induced myoglobinuria. FIET caused only moderate increase in venous lactate and the muscle biopsy showed mild increase of glycogen. In keeping with the site of the block, cycle ergometry showed that glucose infusion decreased the abnormal heart rate and increased peak work rate, just as in McArdle disease. Interestingly, PGM deficiency has also been associated with a congenital disorder of glycosylation (CDG) in a patient with exercise intolerance, increased serum CK, growth hormone deficiency, and first branchial arch syndrome.
Phosphofructokinase Deficiency
Phosphofructokinase (PFK) is a tetrameric enzyme composed of three distinct subunits, muscle (M), liver (L), and platelet (P), which are variably expressed in different tissues. Mature human muscle contains exclusively the M isoform, whereas erythrocytes express both M and L subunits.
In the typical form of PFK deficiency (glycogenosis type VII, Tarui disease), there is a total lack of activity in muscle and partial enzyme deficiency in erythrocytes. The clinical presentation is indistinguishable from myophosphorylase deficiency and is characterized by intolerance to vigorous exercise, with cramps and myoglobinuria. However, PFK-M deficiency may also cause compensated hemolytic anemia, jaundice, and gouty arthritis due to “myogenic hyperuricemia.” In contrast to McArdle disease, patients with PFK deficiency do not experience a second wind and their exercise intolerance appears to worsen, rather than improve, with high carbohydrate intake.
Atypical clinical presentations include hemolytic anemia without myopathy and late-onset fixed weakness. A strikingly different clinical presentation includes myopathy in infancy or early childhood, with respiratory failure and death before 2 years of age. This variant has been reported in several children. Although they all had severe myopathy with PFK deficiency, no molecular defect was documented and the heterogeneity of clinical and biochemical data suggests that we may be dealing with different and possibly diverse molecular etiologies.
In patients with typical muscle disease, laboratory investigations reveal elevated resting levels of serum CK, bilirubin, and uric acid. Reticulocytosis is common, but anemia is not. The FIET is positive but nonspecific. EMG may be normal or show myopathic pattern with irritative features.
Muscle biopsy shows accumulation of subsarcolemmal and intermyofibrillar glycogen. A specific stain for phosphofructokinase allows histochemical diagnosis, which can be confirmed biochemically in muscle homogenate. In most cases, careful observation of the muscle biopsy specimen reveals the presence of an abnormal polysaccharide, which stains intensely with PAS, but is not digested by diastase ( Figure 39.5A ). Ultrastructurally, this abnormal glycogen consists of finely granular and filamentous material, similar to the amylopectin-like polysaccharide that accumulates in branching enzyme deficiency, that is, polyglucosan ( Figure 39.5B ). The deposit of polyglucosan can be explained by the accumulation in muscle of glucose-6-phosphate (G6P), a metabolite upstream of the glycolytic block and an activator of glycogen synthase (GS). This skews the delicate ratio between GS and the glycogen branching enzyme (GBE) in favor of linear synthesis, thus leading to polyglucosan formation.

The gene encoding PFK-M has been assigned to chromosome 1 and the full-length cDNA and the structure of genomic DNA have been reported. Several pathogenic mutations have been identified in Ashkenazi Jewish individuals, who account for most of the patients seen in the United States, and about 20 mutations have been identified worldwide.
Pathogenic mutations in PFKM cause virtual absence of enzyme activity in skeletal muscle and partial PFK deficiency (about 50%) in erythrocytes. Tissues such as liver and platelets express predominantly or exclusively the non-M PFK subunits and are not affected. The lack of clinical cardiomyopathy or encephalopathy is more difficult to explain because PFK-M accounts for over 90% of heart PFK and 50% of brain PFK. Maybe, we should pay closer attention to the heart function of patients with PFK deficiency, as at least one patient had, besides the typical myopathic symptoms, “idiopathic” mild hypertrophic cardiomyopathy and paroxysmal atrial fibrillation.
There is no specific therapy. Glucose administration is not only useless because glucose enters the glycolytic pathway upstream of the metabolic block, but is in fact detrimental because it lowers the blood concentration of alternative fuels, such as fatty acids and ketone bodies.
A 2-year-old boy with the infantile variant of PFK deficiency benefited from a ketogenic diet: there was clear improvement in strength, EMG features, and electroencephalogram (EEG) pattern. Unfortunately, the child worsened suddenly at 35 months of age and died of pneumonia. Still, a ketogenic diet ought to be considered in children with the more severe infantile form of PFK deficiency.
Aldolase Deficiency
Aldolase (ALD) deficiency has been reported in two children with transfusion-requiring nonspherocytic hemolytic anemia, muscle weakness, exercise-induced myalgia, and elevated serum CK. Muscle symptoms became more evident and CK levels were especially high during febrile illnesses. One patient was alive at 4½ years and the other died at 4 years during an episode of myoglobinuria and hyperkalemia. Both children were compound heterozygous for mutations in ALDOA , the gene that encodes aldolase A, the only isozyme present in skeletal muscle and erythrocytes. The vulnerability of patients to fever may be explained by the abnormal thermolability of the mutant enzymes.
Phosphoglycerate Kinase Deficiency
In the second stage of the glycolytic pathway, phosphoglycerate kinase (PGK) catalyzes the transfer of the acylphosphate group of 1,3-diphosphoglycerate to ADP with formation of 3-phosphoglycerate and ATP.
Primary isolated myopathy is not a common manifestation of PGK deficiency, an X-linked recessive disorder most commonly (9 of 33 patients) presenting as nonspherocytic hemolytic anemia and central nervous system disorder (seizures, mental retardation). However, a purely myopathic presentation is equivalent (9 of 33 patients), while isolated blood dyscrasia was reported in 6 patients, and the association of myopathy and CNS dysfunction in 4 patients. However, we may have underestimated this latter group: after describing one patient with myopathy and juvenile Parkinsonism, we found that a second unrelated patient with apparently isolated myopathy but harboring the same mutation in PGK1 also developed Parkinsonism a year later. The lesson here is that we should follow patients with PGK deficiency for a few years before concluding that they have only an isolated myopathy.
Nonetheless, isolated myopathy has been reported in a few patients. These patients complained of exercise intolerance, with cramps and myoglobinuria. Resting serum CK level was inconsistently increased. FIET caused no or inadequate venous lactate increase. EMG was normal. Muscle biopsy showed mild diffuse increase of PAS-positive material.
Human PGK (PGK1) is a monomeric enzyme encoded by a single gene located on chromosome Xq13 and expressed ubiquitously. A testicular isozyme (PGK2) is encoded by a gene on chromosome 19.
The monomeric nature of PGK1 makes it difficult to explain the wide spectrum of clinical phenotypes in PGK deficiency. Different amounts of residual activity in different tissues do not fully explain the clinical heterogeneity. Lack of myoglobinuria in patients with severe hemolytic anemia and encephalopathy may be due to their inability to exercise strenuously, but it is more difficult to explain why patients with pure myopathy do not manifest blood dyscrasia or CNS involvement.
Phosphoglycerate Mutase Deficiency
Phosphoglycerate mutase (PGAM) catalyzes one of the terminal steps of the glycolytic pathway, the interconversion of 2-phosphoglycerate and 3-phosphoglycerate. Human PGAM requires 2,3-biphosphoglycerate as a cofactor and is a dimeric enzyme containing, in different tissues, different proportions of a slow-migrating muscle isozyme (MM), a fast migrating brain isozyme (BB), and an intermediate hybrid form (MB). Normal mature muscle contains predominantly the MM homodimer. Liver, kidney, and brain contain mainly the BB form, and heart contains all three dimer types, MM, BB, and MB. The only other tissue containing substantial amounts of the M subunit is sperm, but there is no evidence of cardiomyopathy or infertility in patients with PGAM deficiency.
The full-length cDNA encoding the M-subunit has been cloned, and the entire gene encoding PGAM-M has been isolated, sequenced, and mapped to chromosome 7p12–7p13.
About 15 patients have been reported, most of them African Americans. All African American patients harbor one common nonsense mutation (W78X), suggesting a founder effect. Different mutations have been found in Italian families, in a Japanese family, and in a Pakistani patient Despite the abundance of PGAM in muscle, we and others have observed exercise intolerance in heterozygous carriers.
All patients had intolerance to intense exercise, with myalgia and cramps, and about half of them had recurrent myoglobinuria. Serum CK level was increased between attacks. FIET caused decreased but not absent venous lactate response. EMG and nerve conduction were normal. Muscle biopsy was normal in most patients, but diffuse or patchy glycogen accumulation was seen in a few cases ( Figure 39.6 ), and about one-third of patients had tubular aggregates, a finding not shared by any other glycolytic disorder and whose pathogenesis remains obscure.

Cycle exercise responses in two patients were markedly different from those seen in McArdle disease: the PGAM patients had virtually normal cycle exercise and oxidative capacity, no second wind, and no improvement of their exercise capacity with lipid or lactate supplementation.
Beta-enolase Deficiency
Like PGM deficiency, beta-enolase deficiency has thus far been reported in a single patient. This 47-year-old man had adult-onset exercise intolerance and generalized weakness but no myoglobinuria. There was no rise of venous lactate with FIET, muscle ultrastructure showed subsarcolemmal deposits of glycogen, and muscle biochemistry revealed an isolated defect of enolase activity, which was 5% of normal. This is because over 90% of muscle beta-enolase is encoded by ENO3 , and the patient harbored two missense mutations in this gene.
Lactate Dehydrogenase Deficiency
Lactate dehydrogenase (LDH) is a tetrameric enzyme composed of two subunits, M (or A), and H (or B), resulting in five isozymes, the two homotetramers M4 and H4, and three hybrid forms. LDH-M predominates in muscle, while LDH-H predominates in heart and in other tissues.
Muscle LDH deficiency (glycogenosis type XI) has been documented in six Japanese families and in two Caucasian patients. The clinical features of this disorder are similar to those of other glycogenoses caused by partial or complete blocks of glycolysis. Some peculiar laboratory and clinical features, however, can offer useful diagnostic clues. During episodes of myoglobinuria, the predictably sky-high levels of serum CK contrast with low levels of serum LDH. FIET causes poor rise of lactate but excessive rise of pyruvate. A characteristic seasonally recurrent erythematosquamous skin rash has been observed in some patients.
Muscle biopsy shows nonspecific myopathic changes, and biochemical study of muscle homogenate reveals a deficit of LDH activity. The sequence of the LDH-M cDNA and the organization of the chromosomal gene have been reported, and the gene has been assigned to chromosome 11p15.4. At least seven different mutations have been reported in patients with LDH-M deficiency.
A Pediatrician’s Perspective
The infantile presentation of PFK deficiency is perplexing because myopathy, often manifesting as arthrogryposis multiplex congenita, is associated with signs of multisystem involvement, including seizures, cortical blindness, corneal opacifications, and cardiopathy. Curiously, none of the infantile cases had evidence of hemolytic anemia, which is difficult to explain. Although the involvement of heart and brain could be attributed to the high proportion of the M-subunit in these tissues, this does not explain why typical adult patients do not have either cardiopathy or encephalopathy.
A more likely possibility is that infantile PFK deficiency may not be due to a molecular defect of PFKM , which has never been documented, but to the defect of another gene, possibly encoding an activator of PFK. Irrespective of the precise cause, ketogenic diet may be useful in these children, based on the experience with one patient.
The defects of terminal glycolysis (PGK, PGAM, and LDH deficiencies) tend to affect adults more than children. However, aldolase A deficiency did affect children and crises were precipitated by febrile illnesses.
Defects of Glycogen Synthesis
Until recently, our knowledge of disorders due to defects of glycogen synthesis was limited to glycogen branching enzyme (GBE) deficiency. However, two novel defects of glycogen synthesis in muscle have been discovered, affecting glycogen synthase (GYS1) and glycogenin (GYG1). Because these new glycogenoses result in the absence rather than the storage of glycogen, they have been called “glycogenoses type zero (GSD 0).” Before describing them, a brief description of glycogen synthesis is in order. It has been known since the 1970s that the richly branched structure of glycogen, which is crucial to its spherical shape (evident in ultrastructural images of glycogen beta particles ( Figure 39.7 ) and to its solubility in the cytoplasm, depends on the synergistic and delicately balanced action of two enzymes. Glycogen synthase (GYS) strands together glucosyl units one to the other in a linear fashion through α-1,4-glycosidic links. When a branch is about 12 glucosyl units long, the branching enzyme (GBE) has to intervene, in two steps. First, it detaches a short linear “twig” of about 4 glucosyl units from a linear chain. Second, it reattaches it in an α-1,6-glycosidic link to the side of a long linear chain, thus starting a new branch that GYS will elongate, and so on. However, one basic question remained unanswered: how does a molecule of glycogen start? What is the “condensation point” that puts together the first glucose molecules? In 1984, William Whelan identified a self-glycosylating dimeric enzyme, glycogenin (GYG), that fits the bill. While defects of the liver isozyme of GYS have been known for many years and attributed to mutations in GYS2 , defects in the muscle/heart isozyme of GYS (gene GYS1 ) and in the muscle isozyme of glycogenin (gene GYG1 ) are relatively recent acquisitions.

Muscle Glycogenin Deficiency
The only reported patient with muscle glycogenin (GYG1) deficiency was diagnosed at age 27 in dramatic circumstances: after exercising, he experienced dizziness and palpitations, went into ventricular fibrillation, and was saved by emergency cardiac defibrillation. In retrospect, he had been unable to keep up with his peers during childhood because of shortness of breath and mild weakness. At age 19, he was dismissed from military duty because of muscle atrophy and EKG abnormalities (an echocardiogram was normal). Family history revealed Charcot Marie Tooth (CMT) type 2 on the paternal side. Neurological examination showed diffuse mild weakness sparing hip and thigh muscles. Serum CK was normal and needle EMG showed slight myopathic changes. The patient was equipped with a cardioverter-defibrillator.
Muscle biopsy showed extreme pallor with the periodic acid-Schiff (PAS) stain, indicating profound glycogen depletion ( Figure 39.8A ). There was marked type I fiber predominance and evidence of mitochondrial proliferation. The endomyocardial biopsy showed large centrally located vacuoles containing PAS-positive material that was digested by diastase ( Figure 39.8B ). Despite the histochemical appearance, ultrastructural examination showed no intravacuolar glycogen but unstructured material together with small vesicles, tubular structures, mitochondria, and lipid droplets ( Figure 39.8C ). Molecular genetic analysis revealed one nonsense and one missense mutation in GYG1 : the missense mutation impaired the autoglucosylation of GYG1, which is essential for the priming of glycogen synthesis.

Muscle Glycogen Synthase Deficiency
Three siblings and two unrelated patients have been described with muscle glycogen synthase (GYS1) deficiency, which involves both skeletal and cardiac muscle. In two of the three siblings, who were born of consanguineous parents, the myopathy was characterized by exercise intolerance noted in childhood, with trouble keeping up with their peers and premature fatigue. The cardiomyopathy was more severe and resulted in a fatal cardiac arrest during exercise in the oldest sibling at 10 years of age. Cardiac involvement was also documented in the other two children, who needed cardioprotective medication. At 4 years of age, the oldest sibling had also had tonic-clonic seizures and received an EEG diagnosis of partial complex epilepsy: however, brain MRI and MRS were normal and the EEG normalized by 6 years of age. In the two younger children, skeletal muscle biopsies stained with PAS showed virtual absence of glycogen in all fibers ( Figure 39.9A ), which was confirmed by electron microscopy (EM) ( Figure 39.9B,C ). There was also a marked predominance of type I muscle fibers and mitochondrial proliferation. Similarly, examination of postmortem cardiac muscle from the oldest patient with hypertrophic cardiomyopathy showed myocyte hypertrophy and lack of glycogen, whereas the content of glycogen in her liver appeared normal. In all three patients, molecular analysis documented a homozygous nonsense mutation in the GYS1 gene.

One other patient, an 8-year-old boy born to consanguineous parents, had a clinical history that was tragically similar to that of the oldest sibling described above: while horsing around at school, he suffered a cardiac arrest and died. There was a history of exercise intolerance. Postmortem examination of skeletal muscle was initially suggestive of a mitochondrial myopathy, with subsarcolemmal accumulation of mitochondria and predominance of type I fibers. However, there was also glycogen depletion and molecular genetic analysis identified a homozygous 2 bp deletion in exon 2 of GYS1 .
The fourth patient was an 11-year-old Japanese girl who, aside from two tonic-clonic seizures (one at age 2 and one at 4), started having repeated episodes of syncope following exercise. Typically, exercise caused myalgia and weakness, making her squat down. This was followed by loss of consciousness for a few hours. Interestingly, FIET failed to raise venous lactate. Surprisingly, however, except for “a mild ischemic finding on exercise electrocardiography,” extensive cardiologic evaluation yielded normal results. Neurological examination showed proximal limb weakness and muscle MRI showed fatty degeneration in glutei and thigh muscles. Muscle biopsy showed glycogen depletion in muscle fibers but not in the endomysium by PAS stain, and lack of histochemical phosphorylase reaction (which depends on the presence of endogenous glycogen). Also, this patient had mitochondrial proliferation. Biochemical analysis of muscle documented the paucity of glycogen (3% of the normal mean) and of GYS activity (2% of normal).
Glycogen Branching Enzyme Deficiency
The glycogen branching enzyme (GBE) catalyzes the last step in glycogen biosynthesis by attaching a short glucosyl chain (about 7 glucosyl units) in an α-1,6-glucosidic link to a naked peripheral chain of nascent glycogen. The newly added twigs are then elongated by glycogen synthase. Hence, GBE deficiency results in the accumulation of an amylopectin-like polysaccharide with fewer branches and longer outer chains than normal glycogen, which is known as polyglucosan (PG). PG is best recognized in tissues with the PAS stain because it is strongly PAS-positive but largely resistant to diastase digestion ( Figure 39.10A,B ). Ultrastructurally, the abnormal polysaccharide consists of filamentous and finely granular material, often associated with normal-looking glycogen particles ( Figure 39.10C ).
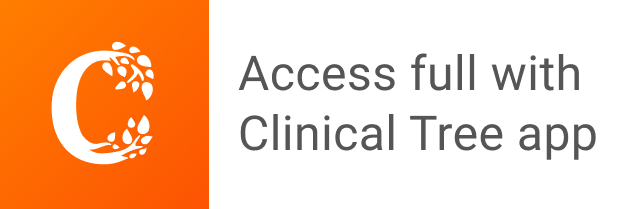