Abstract
Implantable neurostimulators are the tools used by clinicians to execute the various and diverse neuromodulation therapies. Just as it is important for the engineers developing these stimulators to understand the diseases, disorders, and injuries the devices will treat, it is also important for the clinicians using them to understand how these devices operate, the tradeoffs involved in their design, and the capabilities and limitations of the technology. Not only does this mutual understanding allow for proper neurostimulator design on the part of the engineer and optimal prescription and programming by the physician, but it also promotes a dialogue that results in further improvements and breakthroughs in technology and therapy.
Keywords
Clinicians, Design, Engineers, Implants, Neurostimulator
Outline
Introduction 275
Implantable Neural Stimulator Technology 276
Physical Design and Materials for the Stimulator 276
The Neural Interface: Electrodes and Leads 278
Stimulating and Processing Circuitry 279
The Power System 281
Device Communication and Telemetry 283
Sensors for Device Command and Closed-Loop Control 284
Future Directions in Implantable Neurostimulator Technology 285
References 285
Further Reading 287
Introduction
Implantable neurostimulators are the tools used by clinicians to execute the various and diverse neuromodulation therapies. Just as it is important for the engineers developing these stimulators to understand the diseases, disorders, and injuries the devices will treat, so it is important for the clinicians using them to understand how these devices operate, the tradeoffs involved in their design, and the capabilities and limitations of the technology. Not only does this mutual understanding allow for proper neurostimulator design on the part of the engineer and optimal prescription and programming by the physician, but it also promotes a dialogue that results in further improvements and breakthroughs in technology and therapy.
Implantable neurostimulators have their technical roots in cardiac pacing. The first implantable cardiac pacemaker (and arguably therefore the first implantable neurostimulator) was designed by Dr. Rune Elmqvist and implanted on October 8, 1958 by Dr. Ake Senning ( ). Advances in the understanding of disease pathology and the principles of neurostimulation, along with improvements in implantable technologies, have since led to highly reliable and specialized neurostimulators which provide restoration of function for a growing list of neurological diseases and disorders.
The primary function of an implantable neurostimulator is to activate or inhibit the nervous system to augment, improve, or replace function lost to a neurological disease or disorder. As described in detail in Chapter 6 , this modulation of neural activity occurs through the generation of appropriate electric fields within neural tissues. The neurostimulator generates these fields through the application of prescribed currents or voltages to electrodes in contact with or in proximity to the neural tissue.
This chapter describes major aspects of implantable neurostimulator technology, focusing on those elements that most impact clinical practice and device implementation. The topics addressed include physical design and materials for the stimulator; the neural interface electrodes and leads; stimulation and processing circuitry; the power system; device communication and telemetry; and sensors for device command and closed-loop control.
Implantable Neural Stimulator Technology
Physical Design and Materials for the Stimulator
The physical form of the neurostimulator is designed based on constraints, requirements, and ideals from both engineering and clinical realms. The device design must balance the need for a biocompatible, hermetically sealed, and mechanically robust package that is capable of housing all the stimulator components and meeting the clinical demands for minimal invasiveness, conformation to anatomy, facilitation of surgical installment, and device cosmesis.
Most implantable neurostimulators conform to a fundamental organization consisting of three primary components: a centralized implantable pulse generator (IPG), one or more leads, and one or more electrodes (for stimulating and possibly recording). Some of the many commercially available stimulators following this organizational paradigm include all cardiac pacemakers and defibrillators, deep brain stimulators, spinal cord stimulators, vagus nerve stimulators, and cochlear implants. The stimulator houses some or all of the stimulation circuitry (some devices may have external components), and the leads carry the stimulus current to the electrodes, which provide the electrochemical interface to the nervous system. The leads and electrodes are discussed in Chapter 20 .
Stimulator size can range from quite small (Nucleus 24 by Cochlear Ltd, Lane Cove, NSW, Australia, is approximately 6.9 mm thick × 22 mm wide × 50.5 mm long, with much of the length resulting from an external inductive coil ( )) to relatively large (implantable defibrillators with a volume greater than 200 cm 3 have been commercially deployed). The NDI Medical Micropulse stimulator, a fully implantable programmable IPG, has dimensions of 48 mm × 29 mm × 7.5 mm (volume of 8 cm 3 ) and weighs 17 g. The shape of the device will depend on where it is to be implanted. Most neurostimulators are implanted in a subclavicular pocket or in the abdomen and have a familiar flat, rounded shape. The flat shape is designed to minimize the device profile under the skin, and the rounding facilitates surgical insertion and minimizes tissue erosion at the implantation site. For devices that are implanted in locations other than the thoracic or abdominal regions, device shape and size can vary significantly to fit the anatomy relevant to the particular application. For example, cochlear implants must be small enough for implantation in the mastoid process of the temporal bone of the skull (and often have the telemetry/powering coil external to the device can, which is located in a shallow subcutaneous pocket behind the ear). Another device exhibiting a conformational shape is the NeuroPace RNS stimulator (NeuroPace Inc., Mountain View, CA), which is designed for intracranial implantation and therefore has a thin curved profile for conformation to the cortex.
Notable exceptions to the centralized stimulator–lead–electrode design have been developed in response to particular application demands. The Alfred Mann Institute’s Bion (Advanced Bionics/Boston Scientific, Valencia, CA), shown in Fig. 21.1D , is a single-channel stimulator that is fully encapsulated in a glass or ceramic package and is small enough to be inserted via injection into a target muscle for motor functional electrical stimulation (FES) applications or near to a target nerve for applications such as stimulation for migraine headache ( ) or urinary control ( ). At Case Western Reserve University (CWRU, Cleveland, OH) we have developed a distributed motor FES system (see Fig. 21.1 ) that consists of multiple stimulating and recording modules networked together to form an intrabody network. This system, called the Networked Neuroprosthesis (NNP), is designed to provide a scalable and flexible neuroprosthesis platform to meet the variable stimulation needs of those suffering from paralysis or paresis ( ). The NNP design enables recording from and delivery of stimulation to remote sites and implementation of multiple neural implants by the one platform. Retinal implants, which are working toward the restoration of visual function, also depart from the standard form (see Fig. 21.1F ), and are compact systems designed to be implanted on to the retinal surface within the eye ( ).

A standard centralized stimulator consists of two major components: the hermetic package, and the interconnect header for connection of the leads to the stimulator. The primary function of the hermetic package is to keep bodily fluids from reaching the stimulator circuitry and prevent the body from being exposed to the potentially harmful chemicals present inside of the stimulator (especially those in the battery, if present, which is sealed in a hermetic can of its own). Most IPG designs use titanium as the material of choice for the hermetic package, because of its biological inertness, very attractive strength properties, and light weight.
There are several implantable-grade titanium qualities and alloys. Most devices use commercially pure titanium (e.g., grades 1, 2), although the increasing use of transcutaneous powering and recharge systems (see the section below on the power system) is making some of the more power-efficient alloys more attractive (e.g., grade 23). These alloys tend to be more mechanically brittle than pure titanium, which translates into larger bend radius constraints for can molding, but results in less magnetic eddy current loss during inductive power transfer. The increased inductive coupling afforded by these materials can translate into increased power efficiency, decreased device heating, or deeper implantation depths. Other packaging approaches to maintaining power transfer efficiency include using more magnetically transparent packaging material such as ceramic, and locating the powering coil external to the hermetic metal package using hermetic feedthroughs. Ceramic packaging has had an increasing large impact in the packaging of neuromodulation devices, because enclosure of the receiving coil inside the package allows efficient transmission through the package and therefore a smaller package. This approach was pioneered by Erwin Hochmair (1997) and adopted for the Med-El Cochlear implant, and leads to a package size of 33.5 mm × 23.4 × 3 mm. For the external powering coil option, the coil and package tend to be potted together in a biocompatible epoxy (e.g., Medtronic’s Mattrix stimulator (Medtronic Inc., Minneapolis, MN) and the CWRU and NeuroControl Corp. Freehand System (Cleveland, OH) ( ) and IST ( ) motor FES system) or plastic polymer (some cochlear implants). For systems implementing monopolar stimulation, the titanium package (“can”) is often used as the return (common) anode. When this is the case, the stimulator can is often partially coated with (or partially potted in) an insulating material to restrict return path current flow to a particular region of the case which will not be in contact with electrically activatable tissue. This is done to prevent unintended activation of muscle (e.g., the pectoralis muscles if a subclavicular pocket is used) or sensory fibers ( ). The conductors carrying the stimulation current are transferred through the hermetic metal can using glass or ceramic feedthroughs. These feedthroughs insulate the conductors from each other and from the conductive stimulator package and maintain the overall stimulator hermeticity. On the external side of the feedthrough the conductors either connect directly to permanently attached leads or pass into a header which contains connectors for a detachable lead(s) (the header is often cast biocompatible epoxy). The detachable lead(s) is fixed into the connector by either set screws or spring locks. The design of the header and connector takes into account the fact that some assembly is required by the surgeon/implanter: connectors tend to be large for easy handling, and orientation is designed to be unambiguous. Some devices include radiopaque markers in the header to facilitate postimplant identification using radiography. It is also of note that implantable stimulators, leads, and electrodes can only be sterilized using chemical processes (e.g., ethylene oxide), due to the damaging moisture and high temperatures associated with autoclaving.
The Neural Interface: Electrodes and Leads
The portion of the neurostimulator system that is external to the IPG is a solid interconnected structure consisting of the connector(s), the lead(s), and the electrode(s). The basic function of these components is to provide an electrical pathway from the stimulator circuitry to the neural tissue being stimulated. Like the IPG unit, these external components are designed based on restraints and requirements from both engineering and clinical realms. Functionally, the components must provide an isolated current pathway (via the conductors), enable adequate tissue activation and selectivity, adequately conform to the anatomy, and maintain biocompatibility and reliability throughout the device lifetime. This chapter refers to the lead as the insulated conductor(s) between the connector and the electrode. Many device manufacturers and clinicians refer to the lead (as it is used here) and the electrode collectively as the “lead.”
The connector (“pin”) is usually the most proximal (to the IPG) of the external components, although some devices do not use a connector (in which case the lead is permanently connected to the IPG body). In most neurostimulators the connector mates with the IPG header and is secured into place using set screws or a spring-lock mechanism. It should be noted that some stimulators have a short segment of lead permanently connected to the IPG, extending to a more distal mating connector for the lead. Several standard connectors exist, allowing independent selection of the IPG and electrode style to match the needs of a particular patient. Connectors can also simplify and reduce the impact of device replacement procedures, since the lead does not have to be removed. Proper installment of the lead connector into the header is incredibly important to the overall reliability of the neurostimulator, and an improper connection can result in high overall lead impedance and/or improper or ineffective stimulation.
Several other types of connectors exist for some applications. For example, bifurcated connectors allow a single IPG output (usually voltage controlled) to drive electrodes on two leads. Adapting connectors which convert one connector style to another allow the replacement of IPGs with expired batteries while leaving previously implanted leads in place regardless of connector type.
A specialized connector that is very clinically important is the percutaneous connector system. This allows direct access to an implanted electrode lead by a device external to the body via a lead that spans the skin, enabling the patient and clinician to engage in a preimplantation trial/screening period. The screening period allows the clinician and the patient to assess the effectiveness of the therapy before a complete implantation. This approach can allow for finer control of stimulation parameters and serves to reduce cost and invasiveness if it is decided that full implantation is not suitable. Therapies that sometimes utilize this approach include deep brain stimulation (DBS), spinal cord stimulation (SCS) for pain, and motor FES systems. Similarly, neuromodulation therapies often require intraoperative testing to ensure proper electrode placement, which is often performed using an external stimulator set-up similar to the percutaneous system. For example, patients receiving DBS systems for Parkinson’s symptoms are kept under only mild anesthesia while their systems are tested intraoperatively. The leads are connected to a handheld external stimulator, and the patient is evaluated for both positive expressions of the stimulation (reduced rigidity, bradykinesia, or tremor) and negative adverse effects (dystonia, alteration of the visual field) ( ). The patient may also be asked to perform tasks such as drinking from a cup to assess functional outcomes ( ).
The construction of the lead takes into account several factors, including material and mechanical integrity, the safety of the patient, electrical impedance, reliability, and facilitation of surgical implantation. The lead consists of one or more conductors and a material to insulate them from both the other conductors and the harsh body environment. The arrangement of the conductors within the lead can vary significantly, but will generally be multilumen (side-by-side parallel conductors), coaxial (concentric conductors), or helical multifilar design (the conductors are coiled into a long helix). Coiled conductors provide the advantage of reduced stress and torsion during tension, bending, and twisting, thus reducing the likelihood of conductor fracture under these conditions. Multiple strands are sometimes used for each conductor, increasing overall mechanical flexibility and adding redundancy in the event of fracture (most lead fractures occur due to a subclavian crush compression of the lead between the clavicle and the first rib ( )) while maintaining a low lead impedance. The lead is also designed to facilitate surgical insertion, for example by incorporating elements to allow for insertion with a stylus ( ).
Lead conductors must be corrosion-resistant to maintain integrity in the body environment, must have mechanical stability to withstand the twisting, tension, and bending to which they are subjected, and must have a low electrical impedance to help ensure power efficiency. Most neurostimulator leads use composite materials to achieve these properties. Drawn-filled tube ( ) and drawn-brazed strand ( ) filament assemblies are commonly used, and combine a strong, corrosion-resistant material such as MP35N (a nickel alloy) or stainless steel with a highly conductive core material (namely silver). Lead insulators are generally made of robust, biocompatible, flexible polymers such as silicone rubbers or polyurethane. Biocompatible fluoropolymers (e.g., Teflon) are also sometimes used to coat individual filaments within a lead ( ).
Chapter 20 is devoted to the detailed discussion of electrodes, so they are only briefly discussed here. The electrode is the interface between the rest of the neurostimulator and the nervous tissue. Its function is to provide sufficient current to activate or inactivate the target neural tissue with which it is interfaced selectively. Charge can be delivered to the tissue through both capacitive and faradaic mechanisms at the electrode. Unintended nonreversible faradaic reactions (or those that result in unrecoverable charge) can result in damage to the electrode or the generation of reactive species in the tissue. Reversible faradaic reactions can be intended in the design of the electrode, and allow for increased safe charge injection densities by providing the electrode with a pseudocapacitive charge transfer ability. Various metals have been used for neurostimulation electrodes, including gold, stainless steel, platinum, platinum–iridium, and others. The metal chosen for the electrode design is based on biocompatibility, the charge injection required, and surface area constraints.
The physical form of the electrode is designed to fit the target anatomy appropriately and achieve the desired spatial activation and selectivity through its physical shape or electrode configuration (e.g., multiple contacts allowing for multipolar stimulation). Examples of various forms include cylindrical shaft DBS electrodes ( ), electrodes designed to coil inside the cochlea ( ), cardiac pacing and defibrillation electrodes designed for transvenous insertion and anchoring in the atrium or ventricle ( ), peripheral/cranial nerve cuff electrodes ( ), SCS paddle electrodes ( ), epimysial electrodes, and intramuscular electrodes ( ). Some of these electrodes are depicted in Fig. 21.2 .

Stimulating and Processing Circuitry
The stimulus timing, waveform shape, and electrode polarity are of critical importance to the proper activation or inhibition of neural tissue. The stimulation circuitry must be capable of generating the proper waveform on the proper channels with the proper timing for neuromodulation therapy to be effective. Fig. 21.3 shows a standard biphasic stimulation waveform for the activation of neural tissue. During the first phase (the cathodic phase) charge is injected into the tissue, activating nerve fibers (assuming the amplitude and pulse width are sufficient for activation). The second phase (the anodic phase) does not play a role in tissue excitation but serves to discharge the capacitance (and possibly pseudocapacitance if an electrode that utilizes charge transfer-enhancing reversible faradaic reactions is used), making the electrode potential more neutral and stopping potentially harmful faradaic reactions from occurring (e.g., generation of reactive species) ( ). There is typically an interphase interval between these two phases. The duration of the interphase interval is typically chosen to ensure that the efficiency of the cathodic stimulation is not impacted by the anodic phase, and is often 100–400 μs. This waveform is applied to the neural interface (the electrode), and is generated by the stimulation output circuitry of the IPG.

Two general classifications of stimulators are used for neurostimulation: voltage-controlled stimulators (which tend to be used in cardiac pacemakers and deep brain stimulators), and current-controlled stimulators (which tend to be used in cochlear implants and motor FES systems). Both types of stimulators output a waveform of similar shape, but current-controlled stimulators output a current waveform with a shape similar to that in Fig. 21.3 , whereas voltage-controlled stimulators output a voltage waveform with a shape similar to that in Fig. 21.3 . Each method has its own advantages and disadvantages, but both can effectively be used to excite neural tissue. Voltage-controlled stimulators can potentially have simpler circuitry, can be more power efficient than current-controlled stimulators (due to the large compliance voltage that the current stimulators must generate), and are more familiar to the clinical community than current-controlled stimulators (due to the widespread use of voltage-controlled stimulators in cardiac pacemakers and deep brain stimulators). The primary advantage current-controlled stimulators offer is direct control over current injection, the determinant of neuronal membrane depolarization. Current-controlled stimulation produces an injected current that is not a function of the electrode or lead impedance (i.e., will not drift provided the compliance voltage is sufficient) and provides increased consistency in therapeutic settings between individuals in clinical implementation. In voltage-controlled stimulation the delivered stimulus current wanes during the cathodic phase due to the charging of the electrode capacitance. This can result in less predictable activation of the neural tissue due to the variable cathodic current pulse ( ).
In some cases power consumption (see below) may be significantly affected by the simulation waveform. This was explored by using computational models to design novel waveforms to increase the efficiency of neural stimulation.
Multichannel neurostimulation systems are of particular interest. When multiple electrodes are available, unique design configurations are possible. Multichannel systems can potentially implement multipolar stimulation paradigms (bipolar, tripolar, etc.). The simplest and most common of these is the bipolar configuration. In bipolar stimulation, current travels from one peripheral electrode to another peripheral electrode (in monopolar stimulation there is a single return electrode, often the package “can” of the stimulator). Bipolar stimulation results in less current spread than monopolar stimulation, and therefore smaller, more selective activation areas ( ). This unfortunately comes at the expense of higher threshold currents, making bipolar stimulation less power efficient than monopolar stimulation.
Activation of multiple electrodes simultaneously with varied polarities is sometimes referred to as “current steering,” and can be used to increase the selectivity of a given configuration of electrodes by activating tissue that could not be activated by driving the electrodes independently ( ). Multipolar stimulation is commonly used in DBS, SCS, and cochlear implants. Another important aspect of some multichannel systems is their reconfigurability (the ability to assign polarities and stimulation parameters to individual electrodes after implantation). Exact electrode placement is often difficult to achieve and can be complicated by anatomical complexities, the deep nature of the target (e.g., DBS), or the fact that the target is diffuse (e.g., SCS). Electrodes with multiple contacts and creative geometries aid in achieving functional outcomes despite inexact electrode placement. Boston Scientific’s Precision Spectra with 32 contacts and 32 independent power sources is designed to provide coverage over the area of the spinal cord. The ability to assign electrode function has also resulted in improved functional restoration for cochlear implants by reducing current spread and increasing the spatial selectivity of the cochlear electrodes ( ). Similarly, for systems involving multiple discrete electrodes (e.g., motor FES systems), flexibility in electrode assignment allows simpler surgical installation since electrode function can be defined after implantation. Some multichannel systems utilize a time-division multiplexed output configuration, meaning that each stimulation channel is driven sequentially in time by a single stimulator output stage (or subsets of channels are driven sequentially by a single stimulator output stage). This technique serves to reduce the stimulator circuitry required (only a single current or voltage regulator is needed), the instantaneous power demands of the IPG, and the total instantaneous return current flowing back to the return electrode. Large return-path currents can result in unintended activation of tissue and therefore unintended functional outcomes, such as perceived volume changes in early cochlear implants ( ), and inappropriate muscle contracture in motor FES systems. The drawback of multiplexed stimulation systems is that multicontact current steering is not possible if a single stimulator is used.
Another important aspect of implantable neurostimulator circuitry is the implanted processing circuitry. Signal processing occurs by both analog and digital processing elements within the stimulator, and is quite important for systems which include integrated implanted or external sensors (see the section below on sensors for device command and closed-loop control for more detail on sensor types and their role in neuromodulation systems). Analog signal processing usually involves amplification and filtering of a raw sensor output. If a biopotential is being monitored, special circuitry may be implemented to handle stimulus artifact and mitigate the polarization of the sensing electrodes that can occur when the stimulator is operating. The analog signal is usually then digitized and further processed by an onboard microprocessor. Microprocessors are replacing discrete and application-specific logic to become common practice in stimulator design. Not only do they allow careful coordination and timing of events and make communication with external devices more straightforward, but they also make possible the implementation of sensor data-processing schemes and complex control algorithms.
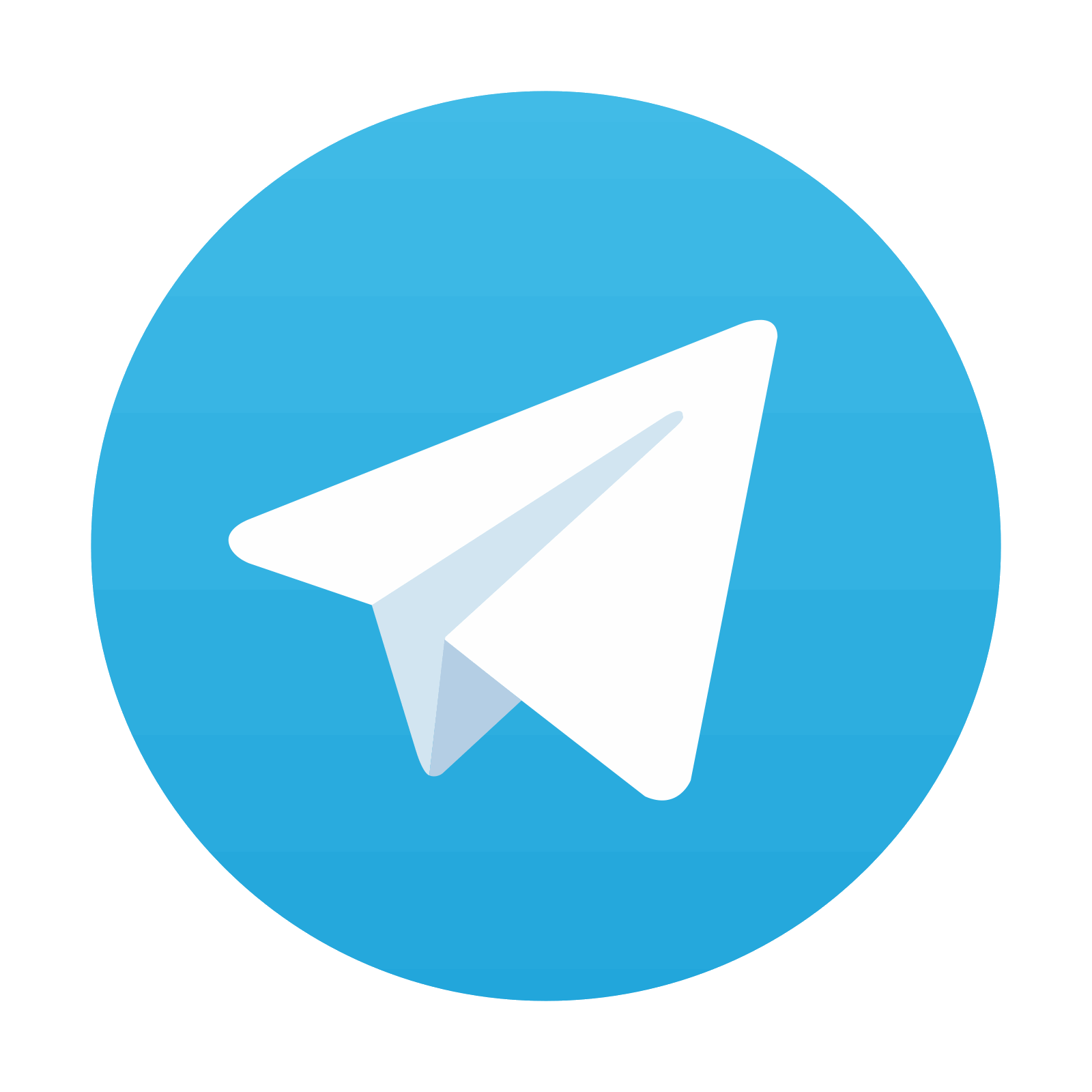
Stay updated, free articles. Join our Telegram channel
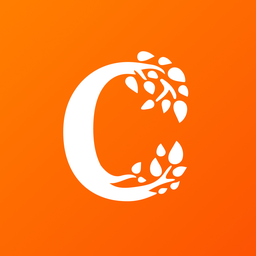
Full access? Get Clinical Tree
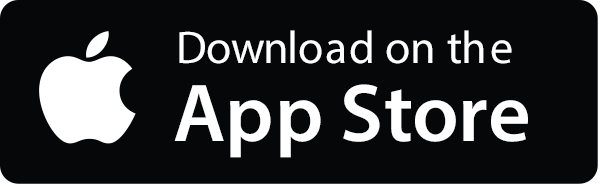
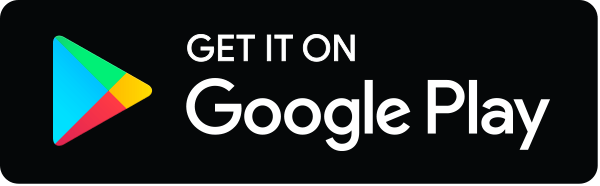