Inflammation
Abstract
The immune response is a complicated system composed of physical barriers, distinct cell types, and inflammatory molecules that serve to protect the brain from foreign pathogens and infections. Neuroinflammation has been demonstrated in both human and experimental models of epilepsy. Cytokines, especially IL-1β, are released in a variety of epilepsies and may induce proinflammatory factors including high mobility group box 1 (HMGB1). Together, these may lead to increased excitability and seizure generation. Similarly, leukocyte infiltration after blood–brain barrier disruption results in ion fluxes and serum protein extravasation that may induce seizure activity. Neuroinflammation can activate distinct signaling pathways, including nuclear factor-κB (NF-κB), which can trigger long-term changes in excitability; these pathways may be regulated by microRNAs (miRNAs). Therefore, limiting proinflammatory cytokine production, leukocyte infiltration, and/or selectively altering neuroinflammation-induced gene regulation may represent therapeutic targets to prevent increased network excitability in epilepsy.
Keywords
Interleukin-1β; IL-1β; leukocyte infiltration; microRNA; neuroinflammation; cytokine; lymphocyte; LPS
Overview
The immune response is an orchestra of physical barriers, distinct cell types, and inflammatory molecules that serve to protect the brain from foreign pathogens and infections. Neuroinflammation has been demonstrated in both human and experimental models of epilepsy. Cytokines, especially interleukin-1β (IL-1β), are released in a variety of epilepsies and may induce proinflammatory factors including high mobility group box 1 (HMGB1). Together, these may lead to increased excitability and seizure generation. Similarly, leukocyte infiltration after blood–brain barrier (BBB) disruption results in ion fluxes and serum protein extravasation that may induce seizure activity. Neuroinflammation can activate distinct signaling pathways, including nuclear factor-κB (NF-κB), which can trigger long-term changes in excitability; these pathways may be regulated by microRNAs (miRNAs). Therefore, limiting proinflammatory cytokine production, leukocyte infiltration, and/or selectively altering neuroinflammation-induced gene regulation may represent therapeutic targets to prevent increased network excitability in epilepsy. The involvement of the brain immune response in epilepsy and the therapeutic potential of targeting specific inflammatory pathways will be discussed in detail in this chapter.
Immune Response in the Brain
The immune response is a complex system used by the body to combat foreign pathogens. It is divided into two main types of response: the innate immune response and the adaptive immune response (Fig. 13.1). Innate immunity is the early, nonspecific inflammatory response that occurs in the brain immediately after an insult or a systemic infection [1]. It is comprised of a physical epithelial barrier to prevent initial infection (eg, the blood–brain barrier), natural killer (NK) cells, and a robust inflammatory response. NK cells are lymphocytes that rapidly detect stressed cells and destroy them. In addition, activated NK cells can produce and release cytokines, small signaling molecules that produce a specific biological response, or chemokines, a type of cytokine that can attract cells to the site of infection/inflammation. Once an inflammatory response is established, more cytokines are recruited along with phagocytes, a type of leukocyte that can recognize and engulf foreign pathogens. Phagocytes can be further subdivided into neutrophils (abundant and fast to respond), macrophages (the most versatile phagocyte), and dendritic cells (phagocytes that are best at activating the specific immune response). After phagocytes break down foreign pathogens, peptide fragments of the pathogen are attached to the major histocompatibility complex (MHC) type II (MHC II), this protein assembly gets transported to the cell surface of the phagocyte, and the cell is now an antigen-presenting cell. They are later recognized in the adaptive immune response.

The immune response is divided into two main categories: innate and adaptive. The innate immune response is the early, nonspecific response that is comprised of a physical epithelial barrier, natural killer (NK) cells, a robust inflammatory response, and assistance by the complement cascade. The inflammatory response is carried out by cytokines and phagocytes (macrophages, neutrophils, dendritic cells). The adaptive immune response is further divided into the humoral (blue) and the cell-mediated (red) response. In the humoral response, activated B lymphocytes differentiate into memory B cells and plasma cells, or effector B cells that produce antibodies. The cell-mediated response is mediated by two types of lymphocytes, helper T cells and cytotoxic T cells. Activated helper T cells differentiate into memory helper T cells and cytokine-producing effector helper T cells. Similarly, cytotoxic T cells divide into memory and effector cytotoxic T cells. Memory cells help “remember” foreign pathogens to accelerate future immune responses to the same pathogen. Effector T cells tag cells for destruction whereas cytotoxic T cells induce apoptosis in targeted cells.
An additional component of the innate immune system is the complement cascade. It is a type of inflammatory response that can be activated through a classical, alternative, or lectin pathway, all beginning with pattern recognition molecules [2]. In the canonical pathway, a C1 complex (composed of C1q and the serine proteases C1r and C1s) fixes antigen–antibody complexes. The complement components 2 (C2) and 4 (C4) are subsequently split, which leads to the formation of the complement component 3 (C3) convertase C4b2b. This promotes the cleavage of C3 and C5, proteins that play a role in inflammation induction. The fragments of complement proteins eventually culminate to form the membrane attack complex (MAC), a protein complex that forms a transmembrane pore allowing free diffusion of molecules into and out of the cell that can lead to target cell lysis.
The innate response may eventually progress into an adaptive immune response, a system that involves a reorganization of the immune system and remembrance of specific pathogens. Although divided into different categories, aspects of the innate immune response, including NK cells and the complement pathway, may aid in the adaptive immune response as well. The adaptive immune response occurs over a longer period of time and is mediated by activated lymphocytes recruited from the blood [3,4]. It is divided two types of response: humoral (mainly mediated by B lymphocytes) and cell mediated (involving T lymphocytes).
B lymphocytes are produced in bone marrow and are covered in membrane-bound antibodies. Once B lymphocytes bind to foreign pathogens and become activated, they start replicating and differentiating into memory B cells and effector B cells. Memory B cells are fairly permanent and “remember” the foreign pathogen to allow for an accelerated and more robust immune response in the future. Effector B cells, on the other hand, produce antibodies (effector B cells that produce antibodies are called plasma cells). These antibodies bind to the foreign pathogen and tag them for destruction by phagocytes, a process called opsonization.
T cells are lymphocytes that mature in the thymus. Helper T cells are activated by binding to MHC II and aid in the activation of B lymphocytes. Activated helper T cells also replicate and differentiate into effector helper T cells (which release cytokines) and memory helper T cells (which improve future immune responses to the same pathogen). Cytotoxic T cells, on the other hand, bind to MHC type I (MHC I), which is present on all nucleated cells. Upon binding to MHC I, cytotoxic T cells become activated and differentiate into memory and effector cytotoxic T cells. Memory cytotoxic T cells aid in future immune responses whereas effector cytotoxic T cells bind to MHC I complexes on cell surfaces and induce cell death through a variety of means, including the release of granzymes (serine proteases that induce programmed cell death) or the exocytosis of perforins to poke holes in the membrane.
T cells are often divided into CD4 expressing (CD4+) or CD8 expressing (CD8+) expressing cells. CD4 is a glycoprotein found on the surface of immune cells that aids in the interaction with MHC II. Therefore, most CD4+ T cells are helper T cells. Similarly, CD8 is a glycoprotein that aids in the interaction with MHC I. Therefore, most CD8+ T cells are cytotoxic T cells.
Is the Brain Immune Privileged?
Immune privilege is a concept that involves keeping adaptive immunity and inflammation highly controlled. The central nervous system (CNS) has been considered immune privileged since the 1920s when it was demonstrated that rat sarcoma transplanted into mouse brain parenchyma survived [5]. Over the years, these studies were furthered by a variety of experiments that showed evasion of immune recognition when bacteria, viruses, or vectors were delivered to the brain parenchyma [5]. In the 1940s, skin grafts transplanted to the brain of nonimmunized animals were successful and did not elicit an immune response [6]. Taken together, these data led to the concept of “immune privilege” in the CNS.
The idea that the brain was an immune privileged site was based on several factors, including the presence of the BBB, the lack of conventional draining lymphatics, a shortage of professional antigen-presenting cells (such as dendritic cells), low levels of MHC molecules, and the presence of many antiinflammatory factors [7]. More recently, however, the concept of an absolutely immune privileged brain has been challenged. Lymphatic vessels and antigen drainage from the brain parenchyma have been characterized [8] and dendritic cells have been found after inflammation [5]. In addition, the CNS has mechanisms for antigen uptake and transport, activated T-cell movement across the BBB, T-cell priming, and immune response cascades. In the injured brain, glial cells are activated, the BBB becomes damaged, MHC II-expressing microglia numbers increase, and inflammation (cytokine and chemokine release) is triggered. Therefore, the idea that brain immune privilege is an absolute concept has faded and been replaced with a relative model of neuroinflammation involving an intricate immune response.
Neuroinflammation and Epilepsy
Neuroinflammation is an integrated response to CNS injury involving several cell types, including resident CNS microglia, astrocytes, and infiltrating leukocytes. When a local inflammatory response is triggered in the brain, the activation of microglia (the resident innate immune cells) and astrocytes causes the release of a number of proinflammatory cytokines, including IL-1β, tumor necrosis factor-α (TNFα), and interleukin-6 (IL-6). In addition, damage to the BBB may allow macrophages, T cells, and B cells to enter the brain and perpetuate the immune response.
Accumulating evidence supports a role of inflammation in the pathophysiology of various types of epilepsy [1,4,9–20]. Inflammation can precede seizures and seizure-induced brain inflammation can persist long after termination of seizures [21]. Microarray analysis revealed that the immune response was the most prominently changed process during all three stages (acute, latent, and chronic) of epilepsy in the hippocampal tetanic stimulation model of epilepsy in rats [22]. Although all cell types are affected by the inflammatory response in the brain, glial cells are particularly involved.
Specific glial inflammatory pathways are chronically activated during the pathogenesis of epilepsy [23,24]. Both astrocytes and microglia were found to be reactive (astrogliosis and microgliosis, respectively) in tissue from epileptic patients [25], including the sclerotic hippocampus of patients with TLE [23,24,26–30] and in focal cortical dysplasia (FCD) [28,31,32], as well as in a number of animal models of epilepsy [24,33–36]. The release of proinflammatory molecules from these reactive cells may alter interactions between glial cells and neurons, particularly targeting astrocytes, which may lead to seizure generation and spread [10]. In addition, reactive astrocytes in the hippocampus of patients with MTLE over-expressed NFκB–p65, a transcription factor known to activate the transcription of numerous proinflammatory genes [23].
Antibody Immune Response
Rasmussen’s encephalitis (RE) is a severe autoimmune form of epilepsy that is usually unihemispheric. It is a heterogeneous disease characterized by neuronal loss [37], microgliosis [37,38], chronic neuroinflammation, vacuolation, lymphatic infiltration [39], intractable seizures and progressive cognitive deterioration. Although RE primarily occurs in children, adolescent and adult cases have been reported.
Specific immunoglobulins (IgGs) were found in neurons from patients with RE, but not from patients with complex partial epilepsy [40]. In particular, circulating antibodies against the subunit 3 (GluR3) of α-amino-3-hydroxy-5-methyl-4-isoxazole-propionic acid (AMPA) receptors have been correlated to RE. Treatment of RE with plasmapheresis or IgG-selective immunoadsorption increased neurological function and reduced seizure frequency, seizure severity, and circulating GluR3 antibodies [41–43]. Therefore, the involvement of autoantibodies against GluR3 have been implicated in the pathology of RE [44–46].
A subset of rabbits immunized with either a GluR3 fusion protein or a portion of the GluR3 subunit developed seizures [47,48]. Antibodies collected from immunized rabbits or humans with RE elicited excitatory currents in cultured neurons [48]. Anti-GluR3 was primarily found on neurons in primary mixed neuronal–glial cell cultures [47]. Although it was thought that anti-GluR3 primarily destroyed neurons [47,49], it was determined that anti-GluR3 can also destroy astrocytes as well [49].
Despite the initial evidence for a role of GluR3 autoantibodies in RE, more recent evidence suggested that these antibodies were not specific to RE but, instead, were found in a variety of epilepsies [50–52]. Furthermore, others have determined that GluR3 autoantibodies were absent or infrequently found in patients with RE [37,51]. Therefore, GluR3 autoantibodies may only play a role in the pathophysiology in a subset of patients with RE.
A growing number of antibodies, including those to glutamic acid decarboxylase (GAD) or voltage-gated potassium channels, have been detected in epileptic patients [46]. Neuronal α7 nicotinic acetylcholine receptor antibodies were found in a subset of patients (2/9) with RE [53]. Two out of ten patients had autoantibodies against the presynaptic protein munc18-1, perivascular accumulation of B lymphocytes (CD20+), and infiltration of plasma cells (CD138+) [54]. These markers have the potential to play a role in the diagnosis and treatment of a subset of patients with RE. For further information about advances in RE and for an overview of other autoimmune epilepsies please see Refs. [55,56].
Cytokines in the Epileptic Brain
Cytokines are small signaling molecules produced by a variety of cells, including macrophages, lymphocytes, and NK cells. Interleukins (ILs), a subset of cytokines, are rapidly secreted from cells in response to a stimulus and bind to their target receptor on a variety of cells. IL-1α, IL-1β, IL-18, IL-12 (active heterodimer is IL-12p70), and TNFα all play a central role in regulating the immune response. IL-6, also known as B cell stimulatory factor-2, stimulates and differentiates B cells into antibody-producing cells (plasma cells). IL-8 plays a major role in chemotaxis and induction of phagocytosis whereas IL-10 inhibits the synthesis of certain cytokines, including IL-2 and TNF-α. Finally, IL-1β mediates a variety of inflammatory responses, including the induction of cyclooxygenase 2 (COX-2) synthesis, and it exerts its biological effects through the IL-1 type 1 receptor (IL-1R1). IL-1 receptor antagonist (IL-1RA) also binds to IL-1R1, therefore acting as a natural inhibitor to IL-1β. Numerous cytokines are cleaved into their active form by cysteine–aspartic proteases (caspases). For example, IL-1β is cleaved into its active form by interleukin-1 converting enzyme (ICE/caspase-1). Increased levels of cytokines can lead to a cascade of pathological events that may contribute to hyperexcitability, excitotoxicity, seizures, and neurological dysfunction (Fig. 13.2) [57].

Cytokines are synthesized and released by glia (microglia and astrocytes) or imported into nervous tissue by blood immune cells, particularly in pathologic conditions associated with a blood–brain barrier (BBB) damage. Excessive levels of cytokines can, in turn, promote both glia and BBB dysfunction, with an impact on neuronal cell excitability and viability. By activating their cognate neuronal receptors, physiological levels of cytokines can modulate voltage-gated channels (VGC) and receptor-operated ion channels (ROC), as well as presynaptic neurotransmitter release. In particular, specific interactions have been reported between IL-1β, TNF-α, IL-6, and glutamatergic or GABAergic neurotransmission. Excessive activation of cytokine receptor signaling in neurons may lead to hyperexcitability and excitotoxicity, thereby contributing to neuronal cell loss, neurological deficits and seizures. Source: Reproduced with permission from Vezzani A, Viviani B. Neuromodulatory properties of inflammatory cytokines and their impact on neuronal excitability. Neuropharmacology 2015;96(Pt A):70–82.
Chemokines are a special class of cytokines that induce chemotaxis, or the movement of cells in response to a stimulus. Members of the C–C cytokine family, including CCL2 (monocyte chemoattractant protein-1, MCP-1), CCL3 (macrophage inflammatory protein 1α, MIP-1α), CCL4 (macrophage inflammatory protein-1β, MIP-1β), and CCL5 (regulated on activation, normal T cell expressed and secreted, RANTES), act as chemoattractants for a variety of cells, including monocytes, NK cells, and leukocytes. Most C–C chemokine receptors (CCR) are G-protein coupled and respond to a variety of chemokines. The CXC chemokine family are separated from the C–C family by a single amino acid (“X”) but its members are also chemotactic. CXC11, for example, acts on its receptor CXCR3 and attracts activated T cells.
Inflammatory effects can be executed through a number of mechanisms. The NF-κB pathway may transcriptionally regulate inflammation and adhesion proteins. Cytokine-induced gene transcription can lead to upregulation of certain adhesion molecules, such as vascular cell adhesion molecule-1 (VCAM-1). Integrins are able to attach to the extracellular matrix of these cells and induce signal transduction cascades. Another mechanism of inflammation regulation is through activating transcription factor-3 (ATF-3), which acts through cAMP-responsive-element binding (CREB) protein to regulate certain inflammatory molecules. The homing cell adhesion molecule CD44 is a glycoprotein that participates in a variety of functions, including the activation, recruitment, and homing of lymphocytes. Mounting evidence has suggested a role for cytokines, particularly ILs, TNF-α, and a variety of chemokines in the pathophysiology of epilepsy.
Human Tissue Studies
Neuroinflammation has been found in human epileptic tissue. Sclerotic hippocampal tissue from patients with TLE exhibited increased levels of transforming growth factor-β (TGF-β) [58], IL-1β, IL-1R1 [24], TNF-α [59], and components of the NF-κB pathway [23,58]. While IL-1β and IL-1R1 immunoreactivity was seen in astrocytes, neurons, and microglia of sclerotic tissue, the majority of staining was found in astrocytes [24]; NF-κB staining, on the other hand, was absent from microglia [23]. Moreover, low levels of AFT-3 and IL-8 genes correlated with high seizure frequency in MTLE patients [60]. Aalbers et al. [61], however, found no difference in cytokine levels between hippocampal sclerosis (HS) and non-HS tissue.
Genetic susceptibility to TLE with HS has been linked to the occurrence of a polymorphism in the IL-1β promoter, specifically at the -511T allele. The frequency of this mutation was higher in genomic DNA extracted from peripheral blood leukocytes in TLE patients with HS than in control DNA [62]. This polymorphism was also linked to sporadic simple febrile seizures (FS) [62–65]. A polymorphism in IL-1RA has also been reported in patients with FS [65].
Patients with FS exhibited increased IL-1β production [66], specifically IL-1β allele 2 (-511) [67], as well as higher plasma IL-1RA and IL-6 levels [68]. These results, however, were controversial because IL-1β is likely to be elevated during any fever. Studies examining cytokine levels in children with febrile illness found no difference in either CSF or plasma levels of IL-1β, IL-10, or TNF-α between children with and without FS [69–72].
Tissue from patients with RE showed astrogliosis but some also showed loss in astrocyte glial fibrillary acidic protein (GFAP) and S100β immunoreactivity around blood vessels or close to the meningeal lining [37]. Activated caspase-3 reactivity, nuclear condensation, and DNA fragmentation suggested that these astrocytes were apoptotic [37]. Similarly, ICE/caspase-1 activity was increased in human tissue from TLE patients [73]. Increased TUNEL-labeled cells as well as enhanced expression of Fas and activated caspase-8 were found in tubers of patients with tuberous sclerosis complex (TSC) compared to control cortex [74]. Taken together, these data suggest that an inflammatory cascade may lead to cell death in a variety of epilepsies.
In a genome-wide study of tissue from patients with TSC, 2501 genes were differentially expressed in resected tubers compared to autopsy controls [75]. These genes include cell adhesion molecules (VCAM-1, integrins, and CD44) and inflammatory response genes (complement factors, CCL2, and various cytokines). Patients with TSC also exhibited increased protein expression of intercellular adhesion molecule-1 (ICAM-1, also called CD54), TNFα, mitogen-activated protein kinase (MAPK), and NF-κB [74]. Reactive astrocytes and activated microglia expressed IL-1β and IL-1R1 in patients with TSC [76].
Similar findings were observed in FCD. Elevated levels of both IL-1β and CCL2 immunoreactivity have been reported in FCD II tissue [32]. In fact, Ravizza et al. [77] found a positive correlation between the number of IL-1β and IL-1R1-positive neurons and seizure frequency and a negative correlation between IL-1RA-positive neurons and astrocytes and the duration of epilepsy in FCD.
Posttraumatic epilepsy (PTE) may result after an injury to the brain. A study of 256 Caucasian adults with moderate to severe TBI found that the CSF/serum IL-1β ratio was higher in patients with PTE than in patients without seizures [78]. Furthermore, the risk for developing PTE increased with the occurrence of a heterozygote (CT) genotype in the rs1143634 single nucleotide polymorphism (SNP) [78].
Human cytomegalovirus (HCMV, also known as human herpesvirus-5 or HHV-5), is a common cause of congenital nervous system infection and may result in mental retardation, deafness, motor deficits, and seizures. Primary human brain vascular pericytes infected with HCMV exhibited elevated levels of proinflammatory cytokines, including IL-8, CXCL11, and CCL5, as well as TNF-α, IL-1β, and IL-6 [79]. The inflammatory response activated by this virus likely played a role in the development of seizures.
Human tissue studies indicate that IL-1β and several other cytokines are upregulated in tissue resected from epileptic patients. Cortical protein levels of IL-1β, IL-8, IL-12p70, and CCL4 were found to be upregulated in patients with various forms of epilepsy, including FCD, encephalomalacia, RE, and MTLE [28]. One major limitation of human tissue studies, however, is that the tissue collected is representative of the endpoint of the disease. To better understand the transition from a healthy brain to an epileptic one (epileptogenesis), appropriate animal models must be used.
Animal Studies
A growing body of evidence has implicated IL-1β and its receptor IL-1R1 in epileptogenesis in a variety of epilepsy models [80–82]. A rapid increase in IL-1β immunoreactivity and mRNA levels were observed after kainic acid (KA)-[34,35,80,82–90], pilocarpine-[24,90], or bicuculline-induced status epilepticus (SE) [80,82]. IL-1β expression was often restricted to or more prominently found in astrocytes after SE. Pretreatment with IL-1β before either intrahippocampal KA-induced [80,83] or bicuculline-induced SE [81,82] significantly reduced seizure latency and increased time rodents spent in seizures [80,83]; this effect was blocked by IL-1RA [80–82]. Increased immunoreactivity [24,83] and mRNA [34,89] levels of IL-1R1, the receptor to IL-1β, were observed in both astrocytes and neurons after KA-induced SE. One study demonstrated that increased expression of the P2X7 receptor was found in the hippocampus and neocortex after intraamygdala KA-induced epilepsy [91]. Antagonism of the P2X7 receptor reduced seizure severity, microglia activation, neuronal injury, and IL-1β release [91].
Higher IL-1RA mRNA levels have been observed after KA-induced seizures [34,84,88,89]. Selective endogenous overexpression of IL-1RA in astrocytes under the control of the GFAP promoter resulted in increased seizure latency, reduced seizure number, and reduced seizure-related c-fos mRNA expression after bicuculline-induced excitability [81]. Similarly, intrahippocampal application of recombinant IL-1RA in mice reduced bicuculline-induced seizures and delayed seizure onset [81]. In vitro isolated guinea pig brain arterially perfused with bicuculline exhibited seizure activity that was associated with overexpression of astrocytic IL-1β [92]. Treatment with the IL-1R1 antagonist reduced the duration of the first seizure and seizure recurrence [92].
A number of other cytokines have been shown to be upregulated at various time points after KA-induced epilepsy, including IL-6, IL-10, IL-12, and TNF-α immunoreactivity [87] and IL-6, IL-10, TNF-α, and suppressor of cytokine signaling 3 (SOCS3, a cytokine-induced regulator of cytokine signaling) hippocampal mRNA levels [34,85,88,89]. Similarly, TNF-α gene and protein levels were elevated in the acute and chronic phases of pilocarpine-induced epilepsy in rats [59,90]. IL-4, IL-6, IL-10, and IL-12 were also elevated in the acute phase after pilocarpine-induced SE [90]. In contrast, young rats exhibited no changes in IL-1RA, IL-6, and TNF-α mRNA [34,89] or IL-6 and TNF-α protein [35] expression levels after intraperitoneal injections of KA compared to control animals.
Polyinosinic-polycytidylic acid (PIC), an immunostimulant structurally similar to double-stranded RNA, injected into mice during embryonic days 12–16 led to increased seizure susceptibility in postnatal day 40 offspring. Specifically, offspring exposed to PIC exhibited increased hippocampal excitability, increased seizure susceptibility, accelerated kindling rate, and diminished sociability [93]. Coadministration with antibodies to IL-6 or IL-1β abolished the effects of PIC. Administration of recombinant cytokines of both IL-6 and IL-1β together, but not individually, had similar effects as PIC [93].
Electrically induced seizures led to transiently increased levels of hippocampal IL-1β, IL-6, IL-1RA, TNF-α, and nitric oxide synthase [82,94]. All of these cytokines returned to baseline levels with the exception of IL-1β, which remained upregulated in the chronic phase [82,94]. Intracerebroventricular injections of IL-1RA reduced the total number of generalized seizures and TNF-α levels after SE [94]. In the amygdala kindling model of epilepsy, however, antiinflammatory cytokine levels (IL-1α, IL-1β, IL-6, IL-10, IL-18, CCL3, and TNFα) remained unaltered 2 and 24 hours after SE [61].
Changes in cytokine levels were also observed after intraperitoneal injections of the convulsant pentylenetetrazol (PTZ). A single dose of PTZ-induced IL-1β mRNA expression in the cerebral cortex, hypothalamus, and hippocampus within hours of treatment [86]. After repeated doses of PTZ in rats, increased mRNA and protein levels of the proinflammatory cytokines IL-1β, IL-6, and TNF-α as well as the chemokine CCL2 were increased in both the cortex and hippocampus [36].
Intrahippocampal KA-induced seizures in the rat led to BBB permeability followed by the concurrent upregulation of CCL2 and immune cell recruitment in the hippocampus [95]. Intraperitoneal injection of KA in rats led to increased levels of CCR5 and its ligands CCL3 and CCL5 in the forebrain [96,97]; reduced expression of CCR5 protected rats from KA-induced seizures, BBB damage, neuroinflammation, and neuronal cell loss [96]. Similarly, increased hippocampal levels of CCL2 and its receptor CCR2 were reported in the pilocarpine model in rats [14,98]. CCR3 and CCR2A gene and protein expressions were downregulated in the hippocampus shortly after pilocarpine-induced SE in mice [99]. CCL2 and CCL3 protein expression decreased but mRNA expression increased after pilocarpine-induced SE.
Rats with FS exhibited transiently elevated hippocampal IL-1β protein levels in astrocytes [100]. IL-1β receptor 1-deficient mice had greater seizure thresholds to experimentally induced FS [101]. Interestingly, infusion of IL-1β in wild-type, but not IL-1β receptor 1-deficient, mice reduced seizure threshold [101]. Comparable results were seen after a viral-like CNS infection in postnatal day 14 (p14) rats caused an increase in IL-1β and made these animals more susceptible to pilocarpine and PTZ-induced seizures as young adults [102]. IL-6-deficient chimeric mice resulted in decreased number of Theiler’s murine encephalomyelitis viral infection-induced seizures [103].
The Tsc1-GFAP conditional knockout (Tsc1GFAPCKO) in mice leads to seizures around 4 weeks of age. At this time, Tsc1GFAPCKO animals exhibited increased CCL2, IL-1β, INF-γ, CXCL10, and IL-6 mRNA levels, decreased CXCL12 mRNA levels, and increased CXCL10 protein levels [104]. In 2-week-old animals, however, only CCL2, IL-1β, and CXCL10 mRNA levels were increased [104]. IL-1β was restricted to GFAP-positive cells. Treatment with rapamycin inhibited the majority of cytokine changes in Tsc1GFAPCKO mice, suggesting the involvement of the mammalian target of rapamycin (mTOR) pathway in the inflammatory response [104].
Several in vitro studies have examined the involvement of IL-1β in cell excitability. The application of IL-1β concurrently with γ-aminobutyric acid (GABA) pulses to hippocampal mixed glial–neuron cultures depressed GABA-evoked currents [105]. IL-1β also decreased the mean amplitude of NMDA-induced outward currents [106] and enhanced NMDA-dependent rises in intracellular calcium [21] in hippocampal neuron cultures. Furthermore, IL-1RA antagonized the effects of IL-1β [21,105,106]. Exposure of preoptic area/anterior hypothalamus neurons in vitro to IL-1β rapidly led to decreased input resistance, hyperpolarization, and increased spontaneous inhibitory postsynaptic potentials (sIPSPs) in 26% of cells [107]. The effects involved type 1 interleukin 1 receptor (IL-1R1), the adaptor protein myeloid differentiation primary response protein (MyD88), and the second messenger ceramide; application of bicuculline abolished the hyperpolarization [107].
Adult rats with genetic absence epilepsy (GAERS—Genetic Absence Epilepsy in Rats from Strasbourg) experience generalized nonconvulsive seizures characterized by synchronous spike-and-wave discharges (SWDs). GAERS rats exhibited increased IL-1β immunoreactivity in reactive astrocytes of the somatosensory cortex [108]. Systemic administration of a specific ICE/caspase-1 blocker to inhibit IL-1β biosynthesis reduced the number and duration of SWDs [108].
Transgenic mice with GFAP promoter-driven astrocyte production of IL-6 (GFAP–IL-6 mice) exhibited enhanced sensitivity to glutamatergic (KA and NMDA) but not cholinergic (pilocarpine)-induced seizures [109]. Increased GFAP levels and astroglial hypertrophy, but not KA-induced neurodegeneration, were evident in GFAP–IL-6 mice [109]. GFAP–TNF-α transgenic mice, however, exhibited similar reactions to KA as wild-type mice [109]. In contrast, Probert et al. [110] demonstrated that overexpression of TNF-α was sufficient to trigger a neurological disorder characterized by ataxia, seizures, paresis, CNS inflammation, and white matter degeneration. Furthermore, transgenic mice with astrocytic, but not neuronal, overexpression of bioactive human transmembrane TNF-α experienced inflammation, seizures, and degeneration [111]. In a separate study, however, targeting TNF-α expression to astrocytes only led to chronic inflammatory encephalopathy [112].
Ceramide, the intracellular signaling product of the enzyme sphingomyelinase, increased intrahippocampal KA-induced seizures and this effect was blocked by preexposure to a sphingomyelinase inhibitor [83]. Furthermore, this process was dependent on phosphorylation of the NR2B subunit of NMDA receptor by the Src family of tyrosine kinases [83]. To support this, a selective NR2B-containing NMDA receptor blocker prevented the proconvulsive activity of IL-1β [21,82,83]. Taken together, these data indicate that ceramide might be a second messenger of IL-1β that exhibits its proconvulsive effects through the NMDA receptor.
High Mobility Group Box 1
HMGB1 is a nuclear DNA-binding protein that can also be released from cells to act as a chemoattractant cytokine. Extracellular HMGB1 can interact with multiple receptors, including the receptor for advanced glycation end products (RAGE), toll-like receptor (TLR) 2, and TLR4 [113]. High levels of HMGB1 have been found in clinical and experimental models of epilepsy [15]. Increased levels of both HMGB1 [114] and TLR4 [60,114] were observed in patients with epilepsy. TLR2, TLR4, and RAGE mRNA and immunoreactivity were elevated in tissue from patients with FCD, TSC, and gangliogliomas (GG) [115].
Immunoreactivity and protein levels of HMGB1 and TLR4 were rapidly increased in both the intrahippocampal KA and bicuculline injection models of epilepsy [114]. Antagonists to HMGB1 and TLR4 reduced both acute and chronic seizures. The application of HMGB1 in wild-type mice prior to KA injections led to a decrease in seizure latency and an increase in number and total time spent in seizures but had no effect in C3H/HeJ (spontaneous mutation in the Toll/IL-1 receptor domain of TLR4) mice [114].
IL-1β induced HMGB1 release in both human [115] and rat [116] cultured astrocytes. Interestingly, in human astrocyte cell culture, HMGB1 was localized in the nuclei but after IL-1β exposure, HMGB1 was also found in the cytoplasm [115]. Furthermore, HMGB1 purified from cells cultured in the presence of IL-1β, interferon-γ (IFN-γ), and TNF-α exhibited prominent proinflammatory activity; this effect was blocked by adding anti-IL-1β antibodies or IL-1RA to cell cultures [117].
Exposure of rat primary astrocytes to HMGB1 resulted in the activation of astrocytes, phosphorylation of MAPK, and increased expression of COX-2 and various chemokines (CCL2, CCL20, CCL3, CXCL1, and CXCL2), but not the inducible form of nitric oxide synthase (iNOS), CCL7, IL-6, TNF-α, or several other inflammatory molecules [118]. The HMGB1-induction of COX-2 expression was blocked by application of either an anti-RAGE antibody or a MAPK inhibitor. A complete mixture of cytokines induced COX-2 and iNOS expression in primary astrocyte culture [114].
Both IL-1β/IL-1R1 and HMGB1/TLR4 cascades are thought to be involved in the pathophysiology of epileptogenesis (Fig. 13.3) [119]. Once activated, IL-1β and HMGB1 bind to their respective receptors and activate the NF-κB pathway [120]. Activation of the NF-κB pathway can then promote chronic neuroinflammation and decreased seizure threshold. In addition, activation of IL-1R1 can lead to phosphorylation of NR2B subunits of NMDA receptors in a ceramide/Src-dependent manner [16,21,83,114,119]. This causes increased NMDA receptor function and NMDA-dependent calcium influx [21], which may also lead to hyperexcitability. Over time, this IL-1R/TLR signaling cascade may lead to chronic inflammation involving elevated cytokine levels, cytotoxic T cell–mediated apoptosis, and overall increased tissue excitability.

(A) Inciting events initiated in the CNS by local injuries, or peripherally following infections, lead to activation of microglia, astrocytes, and neurons in the brain regions involved in the pathological threat. These cells release proinflammatory cytokines such as IL-1β, and danger signals such as HMGB1, thereby eliciting a cascade of inflammatory events in the target cells (ie, neurons and glia) via activation of IL-1R1 and TLR4. Signaling activation in neurons results in a rapid increase of NMDA receptor Ca2+ conductance via ceramide/Src mediated phosphorylation of the NR2B subunit, leading to neuronal hyperexcitability; long-term decrease in seizure threshold results from activating the transcription of genes contributing both to molecular and cellular changes involved in epileptogenesis, and to perpetuate brain inflammation. (B) The effects of brain inflammation originating by the activation of IL1R/TLR signaling contribute to the generation of individual seizures by inducing a decreased neuronal threshold of excitability. Seizure recurrence, in turn, activates further inflammation, thereby establishing a vicious cycle of events that contributes to the development of epilepsy. Source: Reproduced with permission from Vezzani A, Maroso M, Balosso S, Sanchez MA, Bartfai T. IL-1 receptor/Toll-like receptor signaling in infection, inflammation, stress and neurodegeneration couples hyperexcitability and seizures. Brain Behav Immun 2011;25(7):1281–9.
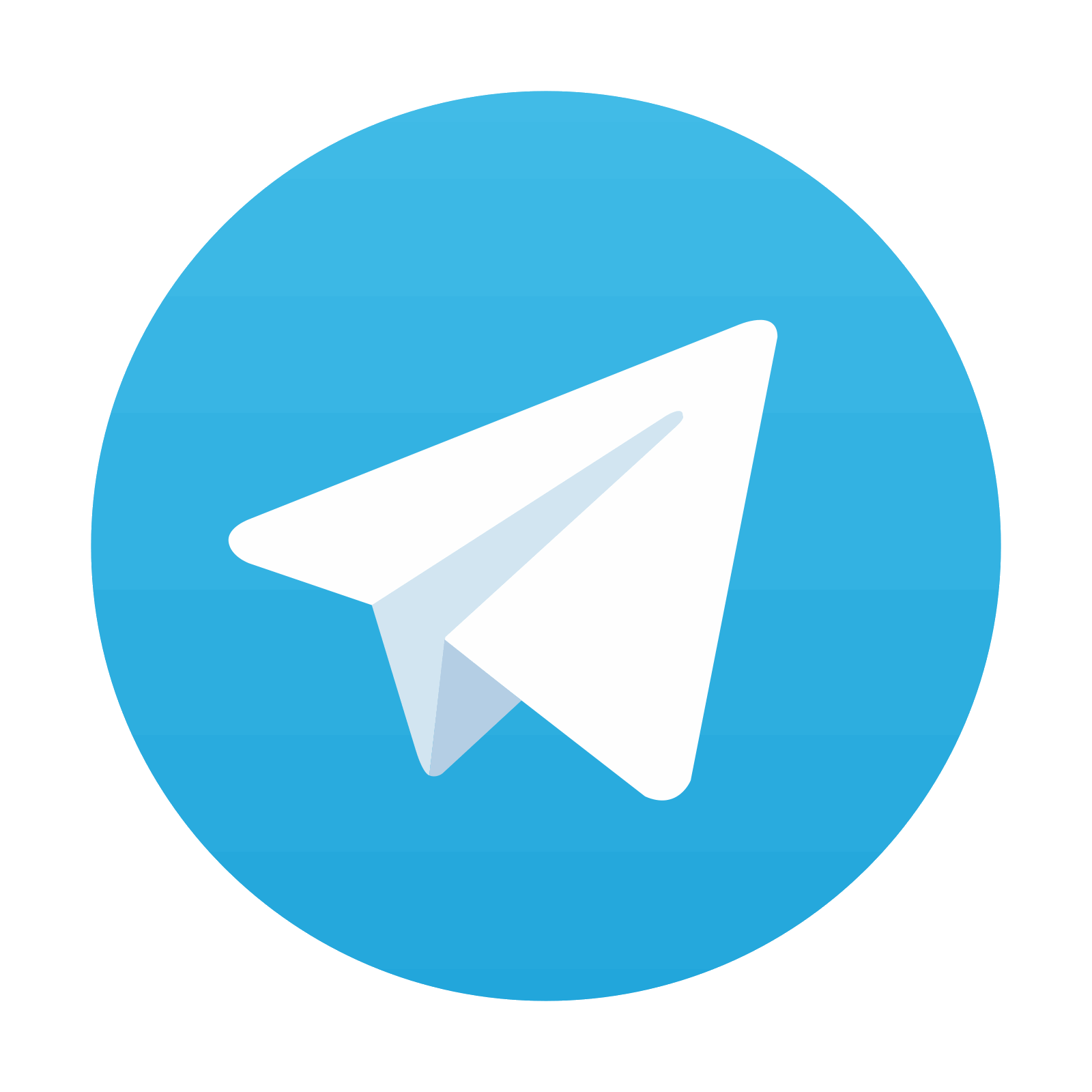
Stay updated, free articles. Join our Telegram channel
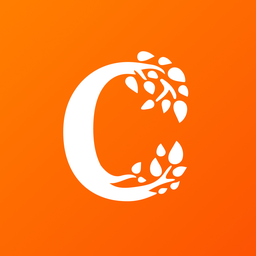
Full access? Get Clinical Tree
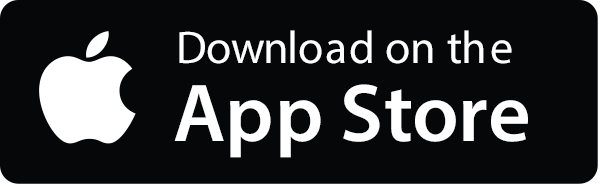
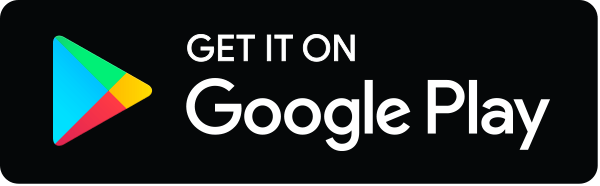
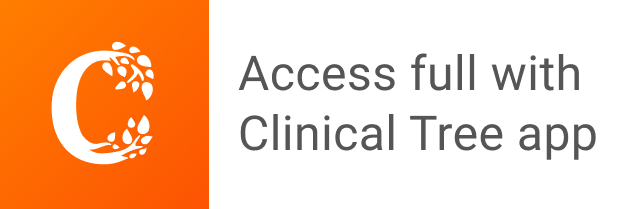